RNA interference (RNAi) was first discovered and characterized in the nematode worm, Caenorhabditis Elegans (Fire et al. 1998). RNAi is a technology that induces gene silencing by double-stranded RNA (dsRNA) that has been processed into short interfering RNAs (siRNAs). In 1995, Guo & Kemphues discovered that downregulation of gene expression in the C. elegans germline can be caused by the injection of antisense RNA.
In 1998, Fire et al. carried out further studies of this phenomenon and named it RNA interference because they considered that it was distinct from antisense inhibition of gene expression. They also described some important characteristics of RNAi, namely that it is induced by double-stranded RNA, that the effect of RNAi is systemic, and that RNAi is heritable.
Following this, RNAi studies were performed on various other systems, which revealed post-transcriptional gene silencing (PTGS) in plants and fungi (Baulcombe, 2004; Cogoni and G. Macino, 1999) and endogenous regulation by miRNAs (Ambros, 2001.)
RNAi is systemic in plants and worms, i.e., the effect can be maintained for three or more generations and can be transmitted with a single sperm (Grishok, Tabara, and Mello, 2000). This systemic feature, which is found in plants and worms, has not been seen in other organisms.
It has been found that dsRNA can induce both post-transcriptional (PTGS) and transcriptional gene silencing (TGS) (Montgomery et al.1998; Grishok et al. 2005; Robert et al. 2005) in C. elegans.
Various components of the gene silencing process have been identified. While some of these components act as initiators, others act as effectors, amplifiers, and transmitters of the process (Agrawal et al. 2003). In 2001, Bernstein et al. discovered an RNase III-like enzyme in an extract of Drosophila.
This enzyme could produce fragments of 22 nucleotides (which was similar to the size produced during RNAi) and is also involved in RNAi initiation. The authors named this enzyme Dicer (DCR) because it could digest dsRNA into uniformly sized small RNAs (siRNA). It is believed that the tandem RNase III domains catalyzes the cleavage by Dicer.
Figure A (see appendix) shows the classical RNAi pathway. This pathway is induced by exogenous dsRNA that is processed into siRNAs by the Dicer complex containing Dicer, the PAZ-PIWI protein Rde-1, the Dicer related helicase Drh-1 and the dsRNA binding protein Rde-4 (Grishok, 2005.)
After Rde-1 is bound to siRNAs, the next step in the RNAi pathway starts. Eri-1 degrades siRNAs, and thereby antagonizes RNAi (Grishok, 2005). The transition between the two steps is mediated by a complex containing Mut-7 and Mut-8/Rde-2. In the downstream step, the RISC complex (containing a single-stranded siRNA, the PAZ-PIWI protein, Tsn-1, and Vig-1) targets a mature mRNA (Grishok, 2005). In addition, another complex containing a RdRP (either Rrf-1 or Ego-1 and possibly Rde-3) is engaged in the target-dependent amplification of the dsRNA. A similar complex containing Rrf-3 amplifies its target and creating competition to RdRP complexes involved in RNAi (Grishok, 2005.)
Figure B (see appendix) shows tandem array silencing. A bi-directional transcription at the locus induces the tandem array silencing. A complex, which likely contains Dicer, a PAZ-PIWI protein, a dsRNA binding protein, and a helicase processes the dsRNA (Grishok, 2005). After the processing, the PAZ-PIWI protein becomes bound to the siRNA. The RdRP complexes, working on the nascent transcript probably uses siRNAs as primers (Grishok, 2005). The siRNA complexes induce H3K9 and H3K27 methylation at the transgenic array and thereby initiate heterochromatin formation (Grishok, 2005). The long dsRNA, which are produced from the array, acts as a substrate for ADARs that successfully compete with the RNAi machinery in the soma, but not in the germline (Grishok, 2005).
In C. elegans, even though the majority of gene silencing due to RNAi occurs at the post-transcriptional level, Grishok et al. 2005 have observed gene silencing at the transcriptional level also. This depends on multiple RNAi pathway genes (rrf-1, rde-1, rde-4, and dcr-1) (Grishok et al. 2005). In addition, transgene silencing depends on the PAZ-PIWI protein Alg-1 and on the HP1 homolog Hp1-2, unlike post-transcriptional gene silencing (Grishok et al. 2005).
Since RNAi has the potential to identify new drug targets and the future design of small molecule drugs, researchers are working on developing siRNA-based drugs (Dykxhoorn & Lieberman, 2006). There are two approaches, which are available for harnessing RNAi in both research and the development of therapeutics. These include siRNA small molecule drugs or shRNA gene therapy (Dykxhoorn & Lieberman, 2006). Song et al., 2003 were the first to demonstrate the ability of siRNAs to protect against disease in a mouse model.
One of the main advantages of siRNA drug development, when compared to more conventional small molecule drugs is that siRNAs can be designed to any host gene or pathogen after knowing the genetic sequences. In addition, they can be tested rapidly (Dykxhoorn & Lieberman, 2006).
In order to harness the ability of siRNAs to be used as drugs, they have to be delivered in vivo into the cytoplasm of cells (Dykxhoorn & Lieberman, 2006). By taking up the double-stranded siRNAs and cleaving the sense passenger strand, the RNA-induced silencing complexes (RISC) are activated (Dykxhoorn & Lieberman, 2006). The RISC is thus left containing the antisense strand to search for mRNAs containing complementary sequences. This will then be targeted for degradation (Dykxhoorn & Lieberman, 2006).
While invertebrate cells readily take up siRNAs, most mammalian cells do not take up siRNAs on their own in a manner, which preserves their activity (Dykxhoorn & Lieberman, 2006). Similarly, siRNAs are not taken up in an efficient manner by dendritic cells and macrophages to activate gene silencing (Dykxhoorn & Lieberman, 2006).
The siRNAs can be introduced in vitro into a variety of cells by using lipid-based transfection (Dykxhoorn & Lieberman, 2006). However, many cells, like primary lymphocytes or hematopoietic stem cells, can be refractory to transfection. In addition, such methods of delivery into cells are not suitable for use in vivo (Dykxhoorn & Lieberman, 2006).
In summary, the most daunting task in developing siRNA drugs is intracellular delivery. Nevertheless, this problem is currently being tackled by effective solutions.
Figure C (see Appendix) shows the cellular pathway for siRNA drug action. This shows the RISC taking up small interfering RNAs that have been introduced into the cytoplasm. After the RISC has been activated, it recognizes a target mRNA bearing a complementary sequence and cleaves it (Dykxhoorn & Lieberman, 2006). Once cleavage of target mRNA occurs, the RISC can be recycled, which seeks and destroys another mRNA (Dykxhoorn & Lieberman, 2006). Two factors, which are related to the potency of siRNA drugs, are the incorporation and stabilization of siRNA in the endogenous RISC complex present in all cells, and the catalytic nature of the cleavage reaction (Dykxhoorn & Lieberman, 2006).
The pharmacokinetics of siRNAs show that unmodified and uncomplexed siRNAs have a very short half-life in the blood. This is the limiting factor of their usefulness (Dykxhoorn & Lieberman, 2006). Another rate-limiting factor is their rapid elimination by the kidneys. They are also degraded slowly by serum RNAses (Dykxhoorn and Lieberman, 2006).
However, despite these limiting factors, some animal disease models show an impressive therapeutic effect, and this promises better effectiveness and improved pharmacokinetic properties for modified siRNA drugs (Dykxhoorn & Lieberman, 2006). Once the active strand of the siRNA is inside a cell, the siRNA, which is incorporated into the RISC, is very stable and the silencing in some tissues may persist for weeks (Palliser et al., 2006; Song et al. 2003; Zimmermann et al., 2006). This persistence of silencing is controlled by two factors: the presence of the target mRNA within the cell, and the rate of cell division of the targeted cell. (Dykxhoorn & Lieberman, 2006).
The following methods help in prolonging the plasma half-life of siRNAs: formation of complexes by siRNAs with other molecules, incorporating it into particles, and by chemical, modification to avoid digestion by exonuclease and endonuclease (Dykxhoorn & Lieberman, 2006). However, these methods will reduce the efficiency of intracellular silencing because of 2 reasons (a) the RNAi mechanism optimally handles unmodified endogenous RNAs, and (b) the unmodified active strand is stable once inside the cell (Dykxhoorn and Lieberman, 2006). Therefore, by minimizing the degree of chemical modification and by restricting it to the linkages or residues that are most susceptible to attack, the efficiency of intracellular silencing can be increased (Soutschek et al., 2005).
In the human body, tissues of the respiratory tract, genital tract, and the eye, have the ability to take up siRNAs either by topical application or by direct injection of naked siRNAs alone or in complexes with cationic lipids used for in vitro transfection (Bitko et al., 2005; Palliser et al., 2006).
The siRNAs have been investigated in the treatment of age-related macular degeneration (ARMD) by intravitreal injection and treatment of respiratory syncytial virus (RSV) infection by intranasal application of naked siRNAs (Dykxhoorn & Lieberman, 2006). Uptake has been demonstrated in the eye and respiratory tract. Three-phase I clinical studies have been completed without any reported toxicity (Dykxhoorn & Lieberman, 2006).
It has also been demonstrated that the cells in the epithelium and lamina propria of the vagina and cervix of the mouse take up siRNA-lipid complexes in a very efficient manner (Dykxhoorn & Lieberman, 2006). This may be because an efficient internalization of the siRNAs takes place on most cells at the mucosal surfaces of the body (Dykxhoorn & Lieberman, 2006). This also suggests that topical siRNA therapies may be more effective and successful.
Since most pathogens use the mucosal surfaces as their main portals of entry, topical siRNAs may be used to prevent or treat intracellular infection by viruses for which there are not many treatment options (Dykxhoorn & Lieberman, 2006). Local siRNA delivery to the lungs has been shown to have a protective effect on nonhuman primates from SARS coronavirus infection (Li et al., 2005).
Viruses not only utilize cellular resources for their own replication but are also capable of modulating cellular pathways, in order to optimize their replication (Berkhout & Haasnoot, 2006). In addition, viruses also have a very close relationship with the cellular RNAi mechanism (Berkhout & Haasnoot, 2006).
Cellular miRNA function can be affected by viral RNAi suppressor factors, which block Dicer or siRNA function. This can especially happen if they cannot differentiate between siRNA and miRNA processing (Berkhout & Haasnoot, 2006).
In 2001, Bitko and Barik demonstrated for the first time that RSV replication could be inhibited in vitro. They used nanomolar concentrations of synthetic siRNAs, which targeted RNAs encoding the viral polymerase subunit P and the fusion protein F.
Other than RSV, RNAi has been able to target many other human pathogenic viruses like poliovirus, HDV, HCV, dengue virus, severe acute respiratory syndrome (SARS) coronavirus, hepatitis B virus (HBV), human rhinovirus (HRV), herpes simplex virus type-1 (HSV-1), influenza A virus, EBV, papillomavirus (HPV), CMV and JC virus (Berkhout & Haasnoot, 2006).
A theoretical concept, that sounds very exciting, is that in the case of viruses with an RNA genome (HCV, SARS, and HIV), therapeutic RNAi would be able to totally destroy the virus from the body. However, this idea is not fully accepted since the viral genome might remain shielded from RNAi, either in the capsid or in the cellular compartments (Grimm & Kay, 2007; Leonard & Schaffer, 2006). In addition, current evidence also suggests that a few of the human viruses could have highly evolved counteractive mechanisms for antiviral RNAi (Grimm & Kay, 2007; Leonard & Schaffer, 2006).
Globally, a large number of people are chronic carriers of the hepatitis virus. Urgent treatment modalities are especially required for HBV and HCV because conventional treatment has little effect on both these viruses, and unlike HBV, there is no effective vaccine for HCV (Grimm & Kay, 2007). Grimm et al., 2006 have engineered double-stranded AAV serotype 8 vectors as a potent anti-HBV.
So far, HIV-1 replication has proved to be very difficult to control due to the ineffectiveness of currently available drugs as well as the high-speed mutation rate of the HIV-1 genome (Lee & Rossi, 2004). Recent studies have shown that RNAi could inhibit HIV-1 replication by targeting viral or cellular genes and could, therefore, be used as a gene-specific treatment option. However, more studies are required to confirm their effectiveness (Lee & Rossi, 2004)
The majority of the studies conducted on HIV used chemically synthesized siRNAs. Shortly before or after a challenge with the HIV-1 virus, these chemically synthesized siRNAs were transfected into cells (Berkhout & Haasnoot, 2006). It was found that although such a transfection effect was only for a short duration, a single application of siRNA could achieve a long-lasting suppression (Berkhout & Haasnoot, 2006.)
Some of the other studies used transient transfection of siRNA-expression vectors (Berkhout & Haasnoot, 2006). It has been found that lentiviral vectors with a Pol III expression cassette can deliver anti-HIV siRNAs into hematopoietic precursor cells (Berkhout & Haasnoot, 2006).
Lentiviral vectors with a Pol III expression cassette are an efficient means to deliver anti-HIV siRNAs into hematopoietic precursor cells (Berkhout & Haasnoot, 2006). In a study by Banerjea et al. 2003 using a SCID-hu thymopoiesis mouse model, transduced human cells were allowed to differentiate in vivo. It was found that the derived mature T lymphocytes were able to resist HIV-1 infection ex vivo.
RNAi may also be effective in the treatment of malignancies like nasopharyngeal cancers, cancers of the head, neck, and cervix, inflammatory diseases like seasonal rhinitis, and inflammatory lung diseases like asthma (Dykxhoorn & Lieberman, 2006.)
Three classes of RNAi targets can be identified for cancer treatment:(a) genes that play a role in tumor-host interactions (b) genes that are part of cancer-associated cellular pathways, and (c) genes that mediate resistance to chemotherapy or radiotherapy (Grimm & Kay, 2007.)
The most effective target would be oncogenes themselves since they are causally linked to malignant transformation. While many oncogenes have been studied in vitro with siRNAs, others are being validated in vivo using RNAi gene therapy (Grimm & Kay, 2007.)
The fusion oncogenes, which are prevalent in lymphoproliferative cancers, are good targets for RNAi therapies since the chimeric mRNA is unique to tumor cells (Grimm & Kay, 2007). During tumor formation, cellular differentiation becomes abnormal. MicroRNAs are important for regulating cellular differentiation. Thus, for the treatment of cancer, drugs based on siRNAs, which mimic or antagonizes endogenous microRNA function may be useful (Croce & Calin, 2005; Hammond, 2006.)
For diseases of the lungs, it is essential to deliver the drug as an aerosol, which will introduce siRNAs into the specific epithelial and immune cells in the lungs. But it is still not known which are the primary cells types that take up naked siRNAs or siRNA-lipid complexes. While earlier studies conducted on animal disease models used hydrodynamic injection (large bolus of siRNAs injected rapidly to cause transient damage to cell membranes in highly vascularized organs) to enable the siRNAs to access the cytoplasm, this technique is not feasible and is dangerous in humans (McCaffrey et al., 2002; Song et al., 2003).
However, therapeutically useful siRNAs can be introduced by catheterizing the vein draining an organ, and thereby, raising the vascular pressure locally (Hamar et al., 2004). In order to direct siRNAs into the deeper cells of the human body, noninvasive strategies have to be used (Soutschek et al., 2004). One such strategy involves the covalent coupling of the passenger strand of the siRNA to cholesterol (Soutschek et al., 2004). This will enable the uptake through ubiquitously expressed cell-surface LDL receptors (Soutschek et al., 2004). This method has been used to target siRNAs to the liver and jejunum. The liver, in particular, is one organ, which is the easiest to target because of its rich blood supply and functions of blood filtering and detoxification.
An alternate way to target specific subsets of cells bearing the specified receptor would be to conjugate siRNAs to other small molecules (either natural ligands for cell-surface receptors or small molecule drugs) (Dykxhoorn & Lieberman, 2006). This would not only reduce the required drug dose but also limit or prevent unintentional toxicity to other nearby cells. SiRNAs can also be delivered to the liver by encapsulating siRNAs into specialized liposomes (Dykxhoorn & Lieberman, 2006). These specialized liposomes are created by using polyethylene glycol, which reduces scavenger cells uptake and enhances the time duration in the circulation. (Dykxhoorn & Lieberman, 2006).
The replication of hepatitis B replicons was shown to be reduced in mice when specialized nucleic acid particles (stable nucleic acid-lipid particles or SNALPs) were used to deliver siRNAs specifically to the liver (Morrissey et al., 2005). In a similar manner, the ApoB expression in nonhuman primates was also observed to be reduced (Zimmermann et al., 2006).
Another strategy is cell-specific targeting, which involves the mixing of siRNAs with a fusion protein (targeting antibody fragment linked to protamine) (Song et al., 2005). By using this strategy, siRNAs can be delivered specifically to a wide range of cells, including primary hematopoietic cells, HIV-infected lymphocytes, and activated leukocytes (Song et al., 2005).
RNAi has found a range of applications for neuroscientists. Most commercial reagents are not able to easily transfect cultured neurons with plasmid DNAs. Compared to plasmids, siRNAs only need to enter cytoplasmic RISC for silencing activity, and are thus are more amenable to standard transfection protocols (Davidson & Boudreau, 2007.)
Commercially purchased or generated siRNAs are complexed with a variety of transfection reagents (Kim et al., 2005). It has been shown that “dicer-ready” siRNAs of 26–27 nucleotides are more potent when compared to the shorter sequences in tissue culture cells (Kim et al., 2005).
Other than whole cells, transfection into spatially restricted areas of neurons has also been achieved. Hengst et al., 2006 have transfected siRNAs targeting RhoA directly into the distal axons of dorsal root ganglia. The authors observed silencing in the transfected axon, but not in the more proximal axons or cell bodies. By this method, the spatial resolution of mRNA transcripts in neurons can be achieved at the subcellular level (Hengst et al., 2006.)
Xia et al., 2002 demonstrated, for the first time, the in vivo delivery to the brain with adenoviruses expressing shRNAs targeting transgenic eGFP, while Makimura et al., 2002 demonstrated plasmids expressing shRNAs against the agouti-related peptide. RNAi (long-term) can be obtained with adenoviruses, lentiviruses, and adeno-associated viruses. These viruses have been engineered to deliver inhibitory RNAs to the nervous system (Davidson & Boudreau, 2007.)
Inhibitory RNAs have also been engineered as treatment strategies for neurological disorders (Davidson & Boudreau, 2007). Various researchers have used RNAi to treat mouse disease models of human neurological disorders including spinocerebellar ataxia type I, Alzheimer’s disease, Huntington’s disease, amyotrophic lateral sclerosis, etc (Dorn et al., 2004, Harper et al., 2005, Makimura et al., 2002.)
Some of the other RNAi vector applications include segregated transgene expression. The biggest challenge in gene therapy is priming the immune system against the newly introduced transgenes. The development of host-mediated immune rejection and vector clearance prevents a stable gene transfer. Earlier methods of controlling these responses, like the use of immunosuppressive drugs or the use of tissue-specific promoters, have had only partial success (Grimm & Kay, 2007).
Brown et al. 2006 tried to overcome neoantigen-specific immunity and induce vector tolerance, based on the role of miRNAs in establishing cell identity. They created a lentiviral vector with a strong neoantigen tagged with a miRNA target, followed by a segregation of transgene expression from the tagged construct.
RNAi technology can also be used to dissect human stem cell biology or to therapeutically modify stem cells (Grimm & Kay, 2007). For this, lentiviruses pseudotyped with the VSV-G glycoprotein seem to be the best vectors (Chang & Sadelain, 2007). Although this technology is still in the early stages, it holds great promise (Grimm & Kay, 2007). Vector-mediated RNAi can also be useful for the genetic manipulation of embryonic stem cells, in order to prevent and treat various diseases (Grimm & Kay, 2007.)
For this, stem cell pools from patients with a specific disease are isolated and clonally propagated. This is followed by the characterization, identification, and correction of the underlying genetic defects (Grimm & Kay, 2007.)
In addition to conventional methods of genetic engineering of animals and plants, vector RNAi technology (animal transgenesis) can also be used as an adjunct and holds great potential in the future (Grimm & Kay, 2007.)
By targeting toxin biosynthesis genes with RNAi constructs, it would be possible to remove plant endotoxins (Agrawal et al. 2005). Another potential application of RNAi includes drug screening and development. This can be achieved by identifying genes that provide drug resistance or genes whose mutant phenotypes are removed by drug treatment (Agrawal et al. 2005.)
Despite their uses, RNAi has potential problems. Like other drugs, siRNAs have unintentional off-target effects (Dykxhoorn and Lieberman, 2006). For example, mRNA cleavage or translational inhibition may unintentionally silence some genes containing sequences with imperfect complementarity (Dykxhoorn and Lieberman, 2006). Off-target effects can also occur by siRNAs mimicking microRNA target recognition and gene silencing (Jackson et al., 2006).
Jackson et al., 2006 described an effective way of reducing off-target effects. This involves the chemical modification of the second residue in the active strand of the siRNA. By this, silencing of the target gene can be achieved, and at the same time, it can suppress unintended off-target effects (Jackson et al., 2006). By using mRNA microarrays, it is possible to survey unintentional off-target changes in mRNA, and they will also help to make any modifications when unexplained toxicity is observed (Dykxhoorn & Lieberman, 2006).
Other than off-target effects, siRNAs could also trigger inflammatory and immune pathways. For example, the interferon response and toll-like receptor (TLR) pathways, which are designed to recognize double-stranded RNAs of invading viruses could be triggered (Sledz et al., 2003).
SiRNA drug resistance can occur, especially with viral infection and cancer, and could be more common with siRNA than other drugs. Synonymous mutations can interfere with the recognition of siRNA and leave the encoded protein untouched (Dykxhoorn & Lieberman, 2006).
There are 3 strategies to deal with this problem. One method is to design siRNAs in a manner that will target sequences that are conserved at the nucleotide level (Dykxhoorn & Lieberman, 2006). Another strategy involves using both siRNA strands, which would target more than one gene or sequence (including drug-resistant variants) (Dykxhoorn & Lieberman, 2006).
The third strategy involves the use of “siRNA cocktails.” This cocktail can be used to attack multiple genes, while for viruses it can be used to target both viral genes and host genes (Dykxhoorn and Lieberman, 2006).
However, by the introduction of exogenous siRNAs into a cell, there could be interference with endogenous microRNA processing and function. This is a potential cause of the toxicity of siRNA drugs. Profusely expressing microRNA-like shRNAs, either using a virus (Lu and Cullen, 2004) or gene therapy vector (Grimm et al., 2006) could interfere with endogenous microRNA nuclear export. At this stage, large intracellular siRNA concentrations might compete for limiting amounts of Dicer or RISC. This factor limits the number of different siRNAs that could be incorporated into a drug cocktail (Dykxhoorn & Lieberman, 2006).
However, even if resistance with siRNA drugs does occur, when compared to other small molecule drugs, siRNA has an important advantage (Dykxhoorn & Lieberman, 2006). While with other small molecule drugs it is difficult to overcome the resistance, with siRNA, it is easy to overcome the resistance by making simple changes in the siRNA sequence (Dykxhoorn & Lieberman, 2006).
To conclude, RNAi is a very efficient and powerful technology of gene suppression and has great potential in the treatment of chronic human diseases. Notably, the ability for systemic delivery, tissue-targeting, and lifelong RNAi persistence make it an attractive therapeutic modality for cancer and antiviral therapy.
However, numerous obstacles still remain before RNAi can become an established therapeutic option. Concerns remain about vector and RNAi safety, siRNA drug resistance, off-targeting, etc. These concerns need to be addressed effectively. In the future, RNAi technology is bound to improve with more efficient and better-designed plasmid or virus-based vectors. These could deliver siRNAs to the tissue of choice and at the required time with minimal side effects.
APPENDIX
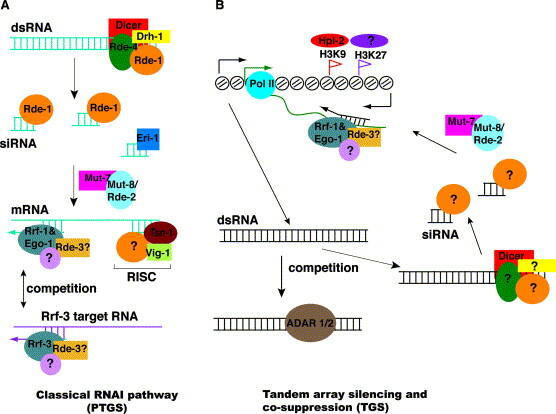
The classical RNAi pathway & Tandem array silencing (extracted from Grishok, A. 2005. RNAi mechanisms in Caenorhabditis elegans. FEBS Letters. 579:26-31.)
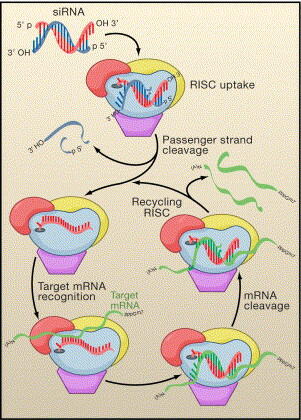
Literature Cited
Agrawal, N, P. V. N. Dasaradhi, A. Mohmmed, P. Malhotra, R.K. Bhatnagar and S.K. Mukherjee. 2005. RNA Interference: Biology, Mechanism, and Applications. Microbiology and Molecular Biology Reviews. 67(4): 1092-2172.
Ambros, V. 2001. microRNAs: tiny regulators with great potential. Cell. 107:823–826.
Baulcombe, D. 2004. RNA silencing in plants. Nature 431:356–363.
Bitko, V, A. Musiyenko, O. Shulyayeva and S. Barik. 2005. Nat. Med. 11: 50–55.
Berkhout, B and J. Haasnoot. 2006. The interplay between virus infection and the cellular RNA interference machinery. FEBS Letters. 580 (12):2896-2902.
Bernstein, E, A. A. Caudy, S. M. Hammond, and G. J. Hannon. 2001. Role for a bidentate ribonuclease in the initiation step of RNA interference. Nature 409:363-366.
Bitko, V and S. Barik. 2001. Phenotypic silencing of cytoplasmic genes using sequence-specific double-stranded short interfering RNA and its application in the reverse genetics of wild type negative-strand RNA viruses. BMC Microbiol. 1: 34.
Banerjea, A, M.J. Li, G. Bauer, L. Remling, N.S. Lee, J. Rossi and R. Akkina. 2003. Inhibition of HIV-1 by lentiviral vector-transduced siRNAs in T lymphocytes differentiated in SCID-hu mice and CD34+ progenitor cell-derived macrophages. Mol. Ther. 8: 62-71.
Brown, B.D, M.A. Venneri , A. Zingale, L. Sergi, L. Naldini. 2006. Endogenous microRNA regulation suppresses transgene expression in hematopoietic lineages and enables stable gene transfer. Nat Med. 12: 585–591.
Croce, C.M and Calin, G.A. 2005. miRNAs, cancer, and stem cell division. Cell. 122: 6–7.
Cogoni, C and G. Macino. 1999. Homology-dependent gene silencing in plants and fungi: a number of variations on the same theme. Curr. Open. Microbial. 2: 657–662.
Chang, A.H and M. Sadelain. 2007. The genetic engineering of hematopoietic stem cells: the rise of lentiviral vectors, the conundrum of the LTR, and the promise of lineage-restricted vectors. Mol Ther. 15: 445–456.
Davidson, B.L and Boudreau, R.L. 2007. RNA Interference: A Tool for Querying Nervous System Function and an Emerging Therapy. Neuron. 53(6): 781-788.
Dorn, G.S, G. Patel, M. Wotherspoon, J. Hemmings-Mieszczak, F. Barclay, J. Natt, P. Martin, S. Bevan, A. Fox and P. Ganju. 2004. siRNA relieves chronic neuropathic pain. Nucleic Acids Res. 32:49.
Dykxhoorn, D.M, J. Lieberman. 2006. Knocking down Disease with siRNAs. Cell. 126 (2): 231-235.
Fire, A, S. Xu, M.K. Montgomery, S.A. Kostas, S.E. Driver and C.C. Mello. 1998. Potent and specific genetic interference by double-stranded RNA in Caenorhabditis elegans. Nature. 391: 806–811.
Guo, S and K.J. Kemphues. 1995. par-1, a gene required for establishing polarity in C. elegans embryos, encodes a putative Ser/Thr kinase that is asymmetrically distributed. Cell. 81: 611–620.
Grishok, A, H. Tabara and C.C. Mello. 2000. Genetic requirements for inheritance of RNAi in C. elegans. Science. 287: 2494–2497.
Grishok, A, J.L. Sinskey and P.A. Sharp. 2005. Transcriptional silencing of a transgene by RNAi in the soma of C. elegans. Genes Dev. 19:25-27.
Grimm, D, K.L. Streetz , C.L. Jopling. 2006. Fatality in mice due to oversaturation of cellular microRNA/short hairpin RNA pathways. Nature. 441:537–541.
Grimm, D, M.A. Kay. 2007. Combinatorial RNAi: a winning strategy for the race against evolving targets? Mol Ther.15: 878–888.
Hamar, P, E. Song, G. Kokeny, A. Chen, N. Ouyang and J. Lieberman. 2004. Small interfering RNA targeting Fas protects mice against renal ischaemia-reperfusion injury. Proc. Natl. Acad. Sci. USA. 101:14883–14888.
Harper, S.Q, P.D. Staber, X. He, S.L. Eliason, I. Martins, Q. Mao, L. Yang, R.M. Kotin, H.L. Paulson and B.L. Davidson, 2005. RNA interference improves motor and neuropathological abnormalities in a Huntington’s disease mouse model. Proc. Natl. Acad. Sci. USA. 102: 5820–5825.
Hammond, A. 2006. MicroRNAs as oncogenes. Curr. Opin. Genet. Dev. 16: 4–9.
Hengst, U, L.J. Cox, E.Z. Macosko and S.R. Jaffrey. 2006. Functional and selective RNA interference in developing axons and growth cones. J. Neurosci. 26:5727-5732.
Jackson, A.L, J. Burchard, D. Leake, A. Reynolds, J. Schelter, J. Guo, J.M. Johnson, L. Lim, J. Karpilow and K. Nichols. 2006. Position-specific chemical modification of siRNAs reduces “off-target” transcript silencing. RNA. 12 (7):1197-1205.
Kennerdell, J. R. and R. W. Carthew. 1998. Use of dsRNA-mediated genetic interference to demonstrate that frizzled and frizzled 2 act in the wingless pathway. Cell. 95:1017-1026.
Kim, D.H, M.A. Behlke, S.D. Rose, M.S. Chang, S. Choi and J.J. Rossi. 2005. Synthetic dsRNA Dicer substrates enhance RNAi potency and efficacy. Nat. Biotechnol. 23:222-226.
Leonard, J.N and D.V.Schaffer. 2006. Antiviral RNAi therapy: emerging approaches for hitting a moving target. Gene Ther. 13:532–540.
Lu, S and B.R. Cullen. 2004. J. Virol. 78: 12868–12876.
Lee, N.S and Rossi, J.J. 2004. Control of HIV-1 replication by RNA interference. Virus Research. 102 (1): 56-67.
Li, B.J, Q. Tang, D. Cheng, C. Qin, F.Y. Xie, Q. Wei, J. Xu, Y. Liu, B.J. Zheng and M.C. Woodle. 2005. Using siRNA in prophylactic and therapeutic regimens against SARS coronavirus in Rhesus macaque. Nat. Med. 11 (9): 944-951.
Makimura, H, T.M. Mizuno, J.W. Mastaitis, R. Agami and C.V. Mobbs. 2002. Reducing hypothalamic AGRP by RNA interference increases metabolic rate and decreases body weight without influencing food intake. BMC Neurosci. 3:18-23.
Montgomery, M.K, S. Xu and A. Fire. 1998. RNA as a target of double-stranded RNA-mediated genetic interference in Caenorhabditis elegans. Proc. Natl. Acad. Sci. USA. 95:15502-15507.
Morrissey, D.V, J.A. Lockridge, L. Shaw, K. Blanchard, K. Jensen, W. Breen, K. Hartsough, L. Machemer, S. Radka and V. Jadhav. 2005. Potent and persistent in vivo anti-HBV activity of chemically modified siRNAs. Nat. Biotechnol. 23:1002-1007.
McCaffrey, A.P, L. Meuse, T.T. Pham, D.S. Conklin, G.J. Hannon and M.A. Kay, 2002. RNA interference in adult mice. Nature. 418: 38–39.
Palliser, D. Chowdhury, Q.Y. Wang, S.J. Lee, R.T. Bronson, D.M. Knipe and J. Lieberman. 2006. An siRNA-based microbicide protects mice from lethal herpes simplex virus 2 infection. Nature. 439:89-94.
Robert, V.J, T. Sijen, J. van Wolfswinkel and R.H. Plasterk. 2005. Chromatin and RNAi factors protect the C. elegans germline against repetitive sequences. Genes Dev. 19:1234-1236.
Sledz, C.A, M. Holko, M.J. de Veer, R.H. Silverman and B.R. Williams. 2003. Activation of the interferon system by short-interfering RNAs. Nat. Cell Biol. 5: 834–839.
Song, E, S.K. Lee, J. Wang, N. Ince, N. Ouyang, J. Min, J. Chen, P. Shankar and J. Lieberman, 2003. RNA interference targeting Fas protects mice from fulminant hepatitis. Nat. Med. 9: 347–351.
Soutschek, J.A, B. Akinc, K. Bramlage, R. Charisse, M. Constien, S. Donoghue, A. Elbashir, P. Geick, Hadwiger and J. Harborth. 2005. Therapeutic silencing of an endogenous gene by systemic administration of modified siRNAs. Nature. 432:173–178.
Xia, H, Q. Mao, H.L. Paulson and B.L. Davidson. 2002. siRNA-mediated gene silencing in vitro and in vivo. Nat. Biotechnol. 20:123-127.
Zimmermann, T.S, A.C. Lee, A. Akinc, B. Bramlage, D. Bumcrot, M.N. Fedoruk, J. Harborth, J.A. Heyes, L.B. Jeffs and M. John. 2006. RNAi-mediated gene silencing in non-human primates. Nature. 441:111–114.