This work entails catalytic hydrogen production which has an extensive application in both mobile application and energy production. Methanol is a high density molecule with excellent H/C ratio and has been reported to be a good hydrogen carrier. In addition, no risk is associated with methanol since it lacks C-C bonds and will thus operate at low temperatures. This work entails developing a catalyst coupled with the construction of a good reformer in the field of catalysis. The first part of this work involved the catalyst Cu/ZrO2/CeO2 which was investigated based on varying copper contents. The CZC catalysts were characterized and their long-term stability and selectivity were investigated, and the kinetic data were determined.
CO is generated in the process and renders Pt electrode of the fuel cell inactive. This led to the use of ammonia which had the disadvantage of hydrazine production. A new type of ammonia cracking catalyst was investigated in this study, which unlike the conventional catalyst is not based on metal. The zirconium oxynitrides was prepared and tested for their activity in ammonia decomposition. A long-term study was carried out on the best catalyst and no hydrazine was detected. The result provides a basis for the further improvement of the catalyst.
Introduction
Energy supply continues to be one of the biggest challenges of our century and remains to be invaluable for our living standards, especially for our mobility and security. However, it could be argued that there are still a large amount of oil and natural gas resources in the world while some oil reserves turned into oil resources since the oil price is becoming higher and higher. The high price of oil has allowed new technical progress for exploration in difficult regions using expensive methods. In the past decade, the USA has continued campaigning against terrorism in the Middle East, and while we continue watching the Ice Age II, a new energy strategy policy is inevitable to provide economic growth and maintain security stability in the energy sector.
Catalysts can be defined as a substance that makes reactions proceed faster without being substantially consumed in the process. Catalyst changes the rate of the reaction at which reactants are converted to products. However, the reaction equilibrium is not affected by the catalysts. In most cases, reactions do not proceed to equilibrium and therefore catalysts work is to influence the rate and the product distribution. A new reaction pathway may be opened by a catalyst by combining it with reactants and conversion proceeding at a faster rate than could have happened in absence of a catalyst. The main characteristic of a catalyzed reaction is a low energy barrier compared to the same reaction when not catalyzed.
The process of catalyzed reaction involve a chemical combination of reactants with reactants molecule, followed by formation of one or more intermediates, and finally into products. The catalyst is released at the end of the reaction and may be used again. This means that, catalysts are recycled although undesired changes may render them less reactive with continued usage. Different catalysts increase the rates of reaction by different orders of magnitude. The activity of a catalyst is a measure of how fast a chemical reaction proceeds. Sometimes, reactants are converted to different products and therefore catalysts should be chosen to favor the major-desired products. Other features of catalysts include stability, which accounts for the catalyst lifetime and regenerability, which describes how well the catalyst is restored to its activity.
The Nature of Catalytic Materials
There are different catalysts in nature. Molecules or ions are found in solution and are the simplest. Biological catalysts (enzymes) are large molecules present in the soft tissues or solutions. On the other hand, industries use robust inorganic catalysts which are capable of withstanding high temperatures. Most catalysts used in large scale natural gas processing or petroleum are metal oxides, metal sulfides, or metals. Solids catalyst offer more advantages than liquid catalysts in that they are noncorrosive, and separates easily from fluid-phase products. In addition, solid catalyst can be used in high temperature and low pressure because the reactants can be in gas phase.
On the other hand, liquid catalysts are more costly since they require high pressures when used in high temperatures. Reactions catalyzed by solid catalysts take place on the surface. To ensure maximum rates of catalytic activity, the surface area per unit volume should be high with the solids having some pores. Various physical properties affecting the catalyst performance include surface area, pore volume, and pore distribution in the surface. These regulate the reactants entry and products molecules out of the pores. Catalysts have different shapes determined by the cost of manufacturing and the requirement of the process. Mostly used catalysts are cylindrical and spherical in shape.
Spherical catalysts (particles) are more advantageous in that they are easily made and transferred into and out of the reactor. Cylindrical particles are formed by intrusion, whereas pellets are formed by compaction and granulation. Many catalysts are composite in nature which consists of support combined with components having catalytic activity or promoters. Some examples of the typical supports include aluminas, silica gel, zirconia, magnesia, zeolites, and carbon. The widely used support is alumina, which is also inexpensive, robust, and stable as well as having the advantage of having wide range of shapes, internal surface areas, and pore size distribution. Metal oxides act as either catalysts or sometimes supports. The catalyst incorporates only a small amount of catalytically active components dispersed on the internal surface of the support (Figure 1).
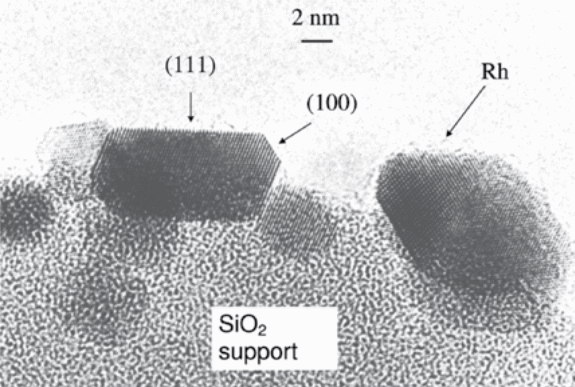
These components are usually very expensive and are therefore present as nanoparticles. Nanoparticles are known to expose a large fraction of their atoms at a surface where the catalysis can occur. Expensive components such as palladium and platinum necessitate technology for their regenerability. A catalyst can either be improved by an added promoter or killed by poison entering with reactant feed stream. Promoters enhance stability, activity, and selectivity on a catalyst.
Catalytic reactors In large scale processes flow reactors are employed in form of fixed-bed reactors, in which the catalyst particles remain fixed in a place whereas fluid phase reactants flow around the particles. Typically the reactors are employed are cylindrically, some large and some small in diameter sometimes surrounded by flowing fluids to supply or remove heat.
Autothermal reactor concept for SRM
The new generation of fuel cells is less sensitive against carbon monoxide and many new catalysts synthesized in the past years have better long term stability and selectivity. However, CO is produced either as a product or by-product and therefore there is need to remove or separate otherwise the performance of the fuel cells will be significantly affected. In the steam reforming process, methanol is converted to hydrogen and carbon dioxide. During this process it depends on the reaction path way at the used catalyst in which explicit intermediates are favored. The main reaction is the Steam Reforming of Methanol (SRM). The Methanol Decomposition (MD) is a parallel reaction which appears negligible at good catalysts.
Many strategies have been invented to lower the amount of carbon dioxide given out in the reformate gases or to purify the hydrogen from the CO gas. For instance, a WGS reactor can be subsequently attached to the reformer or preferential CO oxidation (PROX) can reduce the carbon monoxide content. A study of Ozkara-Aydinoglu et al reported the advantages of a Pt/CeO2 catalyst which has oxygen storage capacity (OSC) and showed an excellent activity at lower temperatures.
Catalysis of Fossil Fuel Production
The major catalytic processes for energy conversion have been developed for petroleum, natural gas, and coal (Table 1). Other processes include power-plant emissions, and conversion of hydrogen in fuel cells. Fractional distillation has been in use in petroleum refineries to yield transportation fuels and petrochemicals. Catalytic conversions of the various fractions are then employed in processing a number of goals; removal of sulfur, nitrogen, and metals from the oil molecules. In addition, reaction of small molecules to give larger molecules, restructuring molecules to improve quality as well as cracking of large molecules to give smaller ones. These processes involve hydrogen gas as reactant, for example, in hydrodesulfurization, hydrodenitrogenation, and hydrodemetallization, which respectively produce H2S, NH3, and solid metal sulfides.
Heterogeneous Catalysis
Many reactions although thermodynamically allowed, do not occur because they are kinetically inhibited. In case the activation energy is too high, the reaction will not occur even if it is an exothermic reaction. In such situations, a catalyst is required to lower the activation energy by providing energetically favorable pathways in which the activation energies of all intermediates steps are lowered compared to the barrier of the gas phases reaction. The first process of heterogeneous catalysis is adsorption. It is always an exothermic reaction of at least one of the gases whose intramolecular bond are weakened or broken and after reacting with other species in several consecutive steps at the surface of the catalyst; the products desorbs into the gas phase and thereby generate the active sites for the catalytic cycle. A catalyst should therefore have a low affinity for the products to enable desorption and high affinity for the reactants.
Kinetic study of SRM over Cu/ZrO2/CeO2 catalysts
In the past decade, considerable attention has been focused on the reduction of the significant emissions originating from mobile sources, such as internal combustion engines. Due to its environmental reasons, the proton-exchange membrane fuel cells (PEMFCs) have gained an increasing importance. While compared to conventional heat engines, fuel application has several advantages including higher efficiency and a more convenient operation, the absence of moving parts and low emission of hazardous compounds. Hydrogen combustion in a fuel cell is regarded as a clean reaction because it releases energy and provides only water as an exhaust material. However, hydrogen is not a natural energy source and must be generated by consuming a large amount of energy, either from natural gas or via the electrolysis of water. Furthermore, for a fuel cell vehicle, the storage and the supply of hydrogen, a volatile and explosive gas, imposes mechanical problems and safety hazards on a commercial level.
Most liquid fuels such as methanol, ethanol, gasoline and diesel have been discussed for onboard reforming. Out of the three, methanol is considered as the most favorable. Although mostly produced from natural gas, methanol can also be obtained from renewable sources, thus lowering the production of carbon dioxide. Moreover, via methanol synthesis from hydrogen and carbon dioxide, methanol can be employed as a storage and transport medium for hydrogen. In addition to its good availability and low boiling point, methanol possesses high hydrogen to carbon ratio (4:1) and contains no carbon-carbon bond, which considerably reduces the risk of coke formation during its reactions. There are various ways of producing hydrogen from ethanol that include decomposition, steam reforming, and partial decomposition.
The decomposition of methanol is a strongly endothermic reaction producing a high CO yield, which makes it rather unsuitable for fuel cell applications. The steam reforming reaction is also endothermic; however, it typically affords a substantial H2 yield of 75%, while maintaining high carbon dioxide selectivity (~ 25%). On the other hand, partial oxidation of methanol is exothermic with a rapid start-up and a dynamic response.
However, the formation of hot spots in the reactor may result in sintering of the Cu particles, which tends to decrease the catalytic performance. The reaction produces considerable lower amount of hydrogen than SRM. SRM has another drawback in that CO form as a by-product which even at low concentration of 100ppm decreases the fuel cell performance by poisoning the Pt electrode. Currently, second stage catalytic reactors are being used to remove CO by the water-gas shift reaction, oxidation or methane formation. However, this CO clean-up unit is rather inconvenient as it occupies a large volume and decreases the efficiency of the fuel cell through hydrogen consumption. In order to eliminate the need for gas purification, high performance catalysts are needed to provide significant methanol conversion and lowest production of CO together with H2 selectivity. However, complete elimination of CO is difficult in SRM conditions.
Considerable studies have been reported in the literature in the past few years on the applications of Cu and Pd-based catalysts for energy of which Cu containing catalysts are clearly preferred because of their high activity and selectivity at lower temperatures. Although Cu catalysts are known to be susceptible to thermal deactivation, their sintering may be substantially reduced by the addition of one or more oxide species, such as ZnO, Al2O3 or Cr2O3. The interaction of Cu and ZnO phases that affect the catalytic activity has been revealed by in situ characterization of Cu/ZnO under SRM activation and operating conditions. Cu/ZnO/Al2O3 catalysts provide high methanol conversions and low CO selectivities which are supported by the kinetic analysis that provide the overall reaction mechanism. Compared to the conventional ZnO or Al2O3- supported Cu catalysts, ZrO2-containing samples have shown increased activities and reduced CO levels for the SRM reaction. The promoting effect of ZrO2 is made possible by an improved reducibility of CuO which tends to increase the dispersion of Cu particles.
Various experiments have demonstrated that ZrO2 prevent the sintering of Cu crystallites under reaction conditions and thus a conclusion that it may be regarded as a stabilizer. Likewise, the application of CeO2, as either a support material or a promoter, has been found to improve the efficiency of Cu-based catalysts. In addition, CeO2 has been reported to increase the thermal stability and the activity of Al2O3-supported Cu catalysts through a synergetic effect. This favors the conversion of CO via the water-gas shift reaction. A major feature of CeO2 is that it has a high oxygen storage capacity, and the partially reduced surface sites formed under reductive conditions produce mobile oxygen, which tends to be of substantial effect on the catalytic performance. For ZrO2-CeO2 supported catalysts, the interaction between the oxide phases, leading to the formation of a thermally stable solid solution, has been reported to increase the mobility of oxygen in both phases.
Hydrogen Production from Ammonia over Zirconia Oxynitride catalyst
Considerable research has shown that Zirconium oxynitrides have a remarkable catalytic activity in the decomposition of Ammonia. In addition, hydrazine is not formed during the extended time on stream. On the quest to replace fossil fuels, particularly in mobile applications, hydrogen is one promising candidate every researcher is considering. Hydrogen usage in generation of power in major available fuel cells appears to be commonly agreed on. However, how to store hydrogen for mobile application is still a controversial debate. Small hydrogen containing molecules can be employed as hydrogen carriers in an on-board reforming process. Other requirements for the process include high-pressure, physisorbed hydrogen, and metal hydrides. There has been much talk on the reforming of fossil fuels based on ecological factors as well as whether it is economic viable.
On the contrary, methanol for example is widely found and can be obtained from biomaterials or from methanol synthesis plants being operated at remote locations where hydrogen is easily available, for instance, from solar energy plants. Therefore, methanol has been voted as a suitable replacement for fossil fuels. However, methanol is linked to a major limitation of formation of carbon monoxide during the reforming process. Carbon monoxide causes fuel cell poisoning and therefore there is a need to remove it in downstream gas purification which reduces the overall efficiency of the reforming process. Another disadvantage is the formation of carbon dioxide which posses ecological concerns. Carbon dioxide is formed in the steam reforming process by carbon-containing hydrogen molecules.
Hydrogen production for mobile application: NH3 cracking over ZrON
Ammonia can be employed as a carrier gas as an alternative to methanol. The large-scale production of Ammonia is well understood and can be operated in less costly places where hydrogen gas is available. The subsequent production of hydrogen by decomposition of ammonia is also well understood. In principle, the process only yields hydrogen and hydrogen gases. However, most catalysts used in this process have a major drawback as there produce significant amounts of hydrazine. Hydrazine is a fuel cell poison similar to carbon monoxide and therefore must be removed in subsequent gas purification unit. There is therefore a need to develop alternative novel catalysts for decomposition of ammonia with low or no production of hydrazine. Hence the limited selectivity of common metal catalysts in production of hydrogen from ammonia originates from the presence of neighboring active sites that leads or facilitates the formation of hydrazine.
The aim of the current work was in two fold. One was the structural and catalytic investigation of novel Cu/ ZrO2/CeO2 catalysts prepared by co-precipitation and its application in energy. The surface area was further increased through employing porous polymer beads as template for the preparation of the solid catalysts. Samples with different Cu loadings were examined for the steam reforming of methanol and their catalytic properties are discussed, including long-term stability as well as CO production. In addition, the reaction mechanism was studied and kinetically analyzed in order to determine the rate constants and the activation energies for the model reactions.
The second aim was to attempt and tailor the selectivity of improved catalysts by seeking novel materials that avoid neighboring active sites. Additionally, this will not only reduce the production of hydrazine but will provide nitrogen atoms from the catalyst bulk for the formation of dinitrogen molecules. For this work, Nitrogen-conducting zirconium oxynitrides were chosen as the promising new ammonia decomposition catalysts that would avoid the presence of neighboring metal sites.
Experimental
Catalyst preparation
The Cu/ZrO2/CeO2 (CZC) catalysts were synthesized from metal sols, prepared from the appropriate amounts of the mixed precursors (NH4)2Ce(NO3)6, ZrO(NO3)2×H2O and Cu(NO3)2×2.5 H2O (all solutions are Aldrich products of > 99% purity), dissolved in 40 ml of distilled water. The total metal content of the resulting sol was 5×10-3 mol. By increasing the pH above 10 through addition of NaOH solution, the co-precipitation of the metal sols as metal hydroxides was achieved. The resulting precipitate was repeatedly washed with distilled water until pH=7 was obtained. The solution was then suspended in a mixture of 5 ml of distilled water and a calculated amount of 90% HNO3 obtained. Depending on the sample composition, the HNO3/metal molar ratio was varied between 1:1 and 1.5:1. Ultrasonic treatment of the suspension for 45 – 60 min resulted in the formation of a transparent sol.
Templating process was carried out by using XAD-16 non-functionalized polystyrene beads (a Sigma product); with a specific surface area of 800 m2g-1 and an average pore diameter of 10 nm. 1.5 ml of the wet beads and 0.005 mol of the metal sol were mixed together and then heated in an oven at 333 K for 2 days in order to ensure solvent evaporation. In a subsequent manner, the beads were dried at 373 K for one extra day and the rinsed thoroughly with distilled water to remove excess deposit from their surface. Later, the beads were dried at 333K for 1 day and then calcined in an oven at 773 K in a N2 stream of 100 ml/min for 2.5 h and then for an additional 8 h in air. After calcinations, the beads turned green-grey as shown in Figure 2.
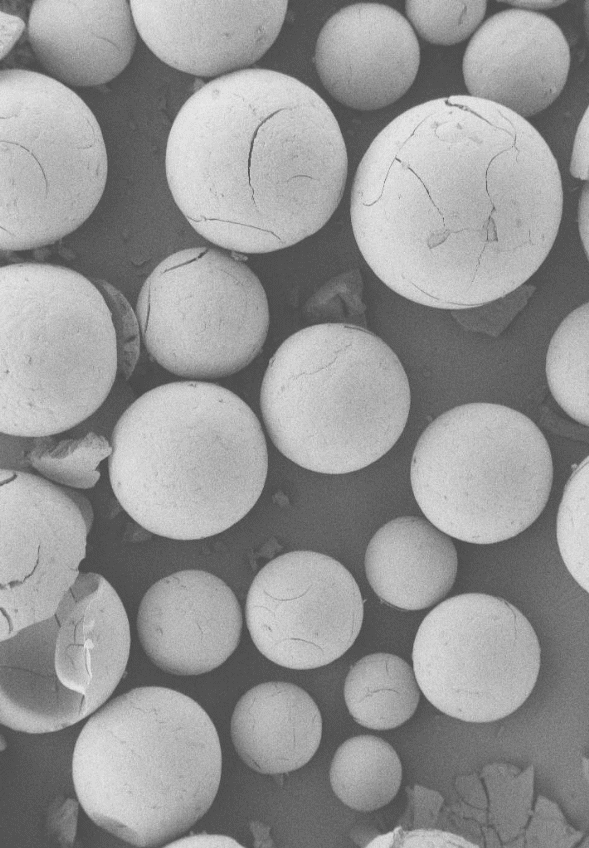
The beads made could be applied as catalysts in fixed bed reactors due to their convenient mean particle diameter (500m) and the regular character of the polymer beads. CZC samples were synthesized by the above method in which the Cu content was 5, 15, 25 and 35% and denoted as CZC5, CZC15, CZC25 and CZC35, respectively. The molar ratio of 1:1 of ZrO2:CeO2 used as the support materials was maintained in each case.
Characterization
X-ray fluorescence analysis (XFA)
Table 1 shows the results of the elementary analysis of the CZC that were performed by a Seiko instrument. According to the result, the amount of Cu detected in the samples was found to slightly lower than the expected value whereas the ratio of 1:1 of the ZrO2:CeO2 was well adjusted.
N2 adsorption-desorption
N2 adsorption-desorption process was employed to determine the specific surface areas of the CZC samples. The temperature of the reaction was set at 77k using a Micromeritics 2375 BET apparatus equipped with a Vacprep 061 degasser. Traces of impurities or water on the surface were removed before measurement at 13 Pa and 395 K for 12 h. The specific surface areas were calculated from the BET equation and the average pore diameters dp were obtained by the BJH method from the desorption branches of the adsorption isotherms.
N2O decomposition
The specific Cu0 surface areas of the samples were measured by N2O decomposition using reactive frontal chromatography (RFC) method. This method is based on the oxidation of the exposed Cu0 surface atoms of a reduced sample by switching the feed from an inert gas stream to a mixture containing N2O as an oxidizing species. The amount of N2O consumed in the reaction is used to determine the surface area of Cu0 as given below (Eq.1)
2Cu + N2O = “Cu2O” + N2
Before measurements, the CZC samples were activated in a stream of MeOH/H2O = 2:1, with a flow rate of 36 cm3min-1 at 523 K. In order to diminish the amount of adsorbed molecules on the Cu surface, the samples were subsequently purged in pure He at 50 cm3min-1 at 523 K for 1 h and then cooled down to 313 K, where the measurements were performed in a 0.5% N2O/He stream of 15 cm3min-1. Further, the catalysts were diluted with boron nitride to provide a significant sample bed of ca. 25 mm height, with a thermocouple positioned directly inside the powder bed. The material was placed onto a quartz frit in a tubular quartz reactor.
For the RFC method the area under the ion current trace (m/e = 28 for N2) was directly used to determine the Cu0 surface area. For the current experiments, however, the same MS signal obtained from the amount of N2 produced for the CZC samples was found to be insufficient for evaluation. Therefore, the amount of N2 formed during decomposition was indirectly estimated from the retarded evolution of N2O with mass to charge of 44 as compared with blank experiments performed with boron nitride. However, earlier investigations for Cu/ZnO samples have revealed a good agreement between the Cu0 surface areas determined by N2O RFC with those obtained from the crystallite size of Copper. The Cu0 surface area was calculated by assuming a stoichiometry of N2O/Cu as 0.5 and a value of 1.47×1019 Cu atoms/m2 determined for the surface density.
X-ray diffraction (XRD)
Ex situ XRD measurements were performed on a STOE STADI P transmission diffractometer (CuKa radiation, Ge monochromator), equipped with a position sensitive detector (PSD, internal resolution 2Q = 0.01°). The Scherrer equation was employed in the calculation of CuO crystallite diameters and the full width at half maximum (FWHM) determined by fitting a Lorentzian profile function to the CuO (111) and (200) diffraction peaks at 2Q = 38.371 and 38.923°, respectively.
Catalytic test reaction
Similar conditions were applied to study the steam reforming of methanol in a fixed bed tubular reactor by utilizing a 3-channel set-up. The set-up ensured that all the three catalysts could be investigated concurrently. An efficient heat transfer in the reactor was further achieved by placing aluminium on a heating block. The temperature of the reactor was repeatedly regulated by PID control of the cartridge heaters located on the interior of the aluminium block. Moreover, time on stream investigations were performed at 523 K and the mass of the catalyst varied between 0.100 and 0.770 g, depending on the Cu content, in order to keep the amount of CuO constant for all samples (0.0225 g).
Other measurement such kinetic analysis entailed increasing the mass of the catalyst by a certain factor such as 2.7 which corresponded to a CuO content of 0.0584 g for each sample, to achieve full conversion in the temperature range investigated (503-573 K). A stainless steel grid was used to support the catalyst inside the reactor. For flow conditioning, inert Pyrex beads (d = 500 mm) were placed below and on top of the catalyst bed. The reactant mixture of MeOH (HPLC grade, 99.9% purity) and distilled water, with a molar ratio MeOH/H2O = 1:1, was introduced into the reactor by means of a Dionex HPLC P 580 pump. All the reactants were completely degassed before use at 20kPa. The instruments were assembled such that the time-on-stream measurements had a flow rate for a single channel at 0.07 cm3min-1, whereas for kinetic investigations, it was varied between 3.33×10-3 and 0.67 cm3min-1.
Since catalysts aging effects were more pronounced in this work, kinetic measurements were performed by employing fresh catalysts to eliminate the effect of aging. In addition, experimental data for time on stream were collected after the fifth day. Steady state conditions were achieved by collecting the experimental data at low flow rates, for instance, 60 minutes after the flow rate had been adjusted. Activation of the catalysts in situ was carried out before measurement. Activation was done in MeOH/H2O = 1:1 stream of 0.07 cm3min-1 at 523 K for 15 hours. The effluent gases mainly consist of the main products, which are H2 and CO2 and a minor amount of CO as the reaction products. The products together with unreacted methanol and water were passed through a condenser at 273 K, which removed most of the liquid from the product gas stream.
The condensed reactant mixture was completely removed by employing an additional condenser at 253 K, and the liquid composition analyzed using a gas chromatography, which was equipped with a thermal conductivity detector (TCD), operated with a 50m x 0.53 mm CP-Wax column at 363 K. The composition of the dry product gas mixture was determined on-line, by means of a Varian 3800 GC with a TCD. Helium was used as a carrier gas and separation was achieved by using a 25 m x 0.53 mm CarboPLOT P7 column at 304 K. Steam was analyzed quantitatively using a calibration gas mixture containing 0.5% CO, 4.5% N2, 25% CO2 and 70% H2. Under the present experimental conditions, no evidence for the formation of the by-products methane, methyl formate, and dimethyl ether was found.
Results and Discussion
Catalyst characterization and long-term stability
Table 1 gives the summary of the results that were obtained for from the CZC samples N2 adsorption-desorption and N2O decomposition:
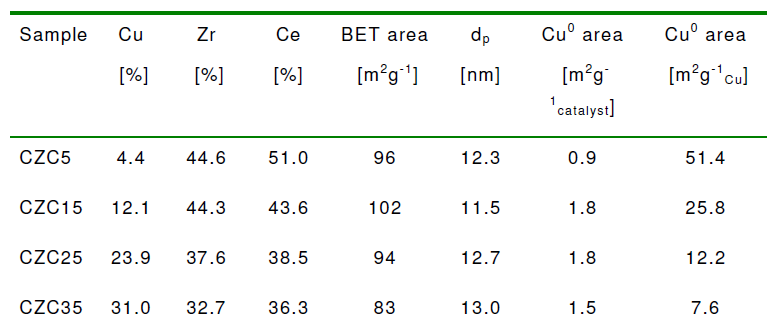
For our results the BET surface areas obtained for the CZC samples were considerably higher than those reported for ZnO supported Cu catalysts. As shown in Table 1, the values for the average pore diameter were very close for each sample. Although the Cu0 specific surface areas for the CZC materials proved to be lower than expected from the internal surface areas and the Cu contents, the values exhibited a similar trend as the BET results. However, an increasing Cu content resulted in a decreasing Cu0 surface area roughly in agreement with an increasing Cu particle size. In addition, we found that the net decrease of the Cu0 specific surface area with increasing Cu content, observed for CZC15, CZC25 and CZC35, is in good agreement with earlier results reported in the literature for supported Cu catalysts.
According to the XRD pattern in Figure 1, the characteristic Cu0 peaks could be easily distinguished for samples with highest Cu contents as revealed. There were no Cu0 signals for CZC5 and CZC15 which was attributed in part as the result of lower Cu loadings. Furthermore, if the Cu crystallite size for the above samples is too small it will not be detected by XRD, as a result of pronounced line broadening. The broadening of the peaks also renders it difficult to establish whether the Zr, Ce and O atoms are arranged in one single mixed phase or in separate phases. The above reason has rendered it difficult to determine the shape of the corresponding crystal structures. The Cu crystallite sizes obtained from the diffraction peaks for CZC25 and CZC35 were 12.4 and 15.3 nm, respectively.
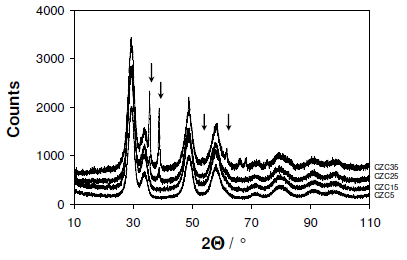
In order to make a comparison between the long-term stabilities of the CZC catalysts, the methanol conversions obtained during a continuous operation of 16 days under standard conditions were plotted as a function of time-on-stream as depicted in Figure 2 below.
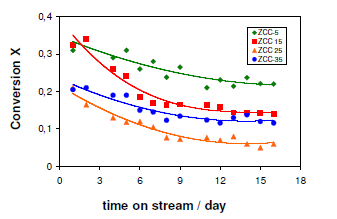
It is clear from Table 1 and Figure 2 that there is no linear correlation that exists between the catalytic activity and the Cu surface area. Evidently, the activity of the CZC samples is not only determined by the Cu surface area, but also by the particular microstructure copper particles which may be similar to the microstrain in Cu/ZnO catalysts. After 5 days the catalytic activity considerably diminished although at different extent. There was no systematic variation with the Cu content displayed as a factor of diminishing of Cu content. The conversions determined after 5 days approached a constant value, except for CZC5, which exhibited a significant decrease. The CZC samples containing more than 5% Cu contents displayed significantly long term stabilities after the initial period of deactivation. These seemed suitable for the extended operation.
The relatively poor thermal stability of CZC5 may be linked to the small Cu particle size, considering that small particles tend to be more susceptible to thermal sintering under reaction conditions, even at temperatures lower than 573 K. For comparison purposes the CZC samples containing no copper were prepared using the procedure described above. The as prepared new material did not exhibit any detectable activity in the steam reforming of methanol under the reaction conditions employed. Hence, the differences observed for the CZC catalysts in the steam reforming of methanol can be mainly attributed to the bulk and surface properties of the Cu phase in these materials.
The carbon monoxide was determined during the above measurements is depicted in Figure 3 below.
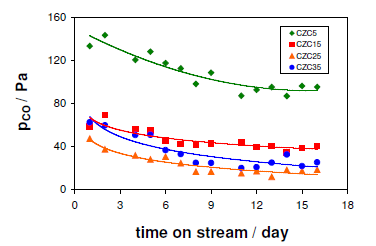
As previously mentioned, the sample masses were adjusted to provide the same Cu content. For this reason, the mass of CZC5 considerably exceeded those of the other samples, and this may accounted for the amount of CO detected for CZC5 being substantially higher than those for the samples with higher Cu contents. In addition, after five days of pronounced decrease the carbon monoxide levels stabilized and did not exhibit any significant difference. This finding is consistent with the results of N2O decomposition, indicating that the active Cu contents of these samples were very similar.
Here, it may be concluded that from Figure 2 and 3, the increase in Cu content from 5 to 15 percent has a beneficial effect on the catalytic performance of CZC through the long-term stability and suppressing of the carbon dioxide production. Further observations were that increase of the Cu loading proved to be less important for the stability of the catalyst although it had a beneficial consequence of low carbon monoxide production. However, the carbon monoxide production depends on the conversion of methanol as the activity order of the CZC samples is in correlation with the order of the CO levels throughout the entire time on stream (Figure 3). Accordingly, under the present experimental conditions, the samples with increased Cu contents may be regarded as more efficient catalysts for SRM, with respect to the formation of CO in particular. Considering that the CZC catalysts are porous materials and the methanol conversions in Figure 2 displayed no systematic variation with either the Cu loading or the Cu0 surface area, the effect of mass transport limitations on the catalytic performance was additionally investigated.
Since the CZC samples were not uniform in size as previously observed, thus each material three fractions with different particle diameters could be obtained by sieving method. Moreover, a Cu/CeO2 sample containing 25% of Cu, prepared by the same method, was found to slightly deteriorate during SRM, as a small amount of the original catalyst beads (3.3%) was transformed into a fine powder after reaction. On the other hand, the Zr-containing beads of the CZC samples remained essentially unchanged after catalytic investigations, which confirm that ZrO2 is an important structural stabilizer.
Kinetics of the catalysts
The reaction to be included in the kinetic model of the methanol steam reforming process is still under controversial debate. Earlier studies have suggested that the kinetics could be sufficiently described by using only one or two of the possible overall reactions while assuming that the others were either at equilibrium or their rates were negligible. Initially, the formation of carbon dioxide by the direct reaction of methanol and steam was also been proposed. Methanol decomposition was involved in the reaction mechanism in certain studies, whereas in other cases, it was regarded as insignificant. A sufficient kinetic model for the steam reforming of methanol on a commercial Cu/ZnO/Al2O3 catalyst, which includes methanol decomposition (Eq.1) and the SRM process (Eq.2), has been recently suggested.
Further investigations on SRM revealed that the above model may also be applied to other catalysts including Zr- or Ce-containing systems. For the present study, it was found that a kinetic evaluation performed by including MD in the reaction scheme afforded a better fit to the experimental data. Carbon dioxide formation was generally observed at high methanol conversions and along contact times indicating that carbon is a secondary product formed by the reverse water-gas-shift (RWGS) reaction. For the CZC samples, kinetic analysis suggested that the amount of CO produced by MD was considerably lower than that formed by the RWGS reaction and therefore the reaction scheme was simplified by regarding MD as an irreversible reaction.
For the most active catalyst (CZC35) investigated at the highest reaction temperature (573 K), the Thiele modulus was calculated to be 0.594, from which the mass transfer factor (the actual reaction rate as referred to the ideal reaction rate without transport limitation) was 0.977, indicating that the mass transport limitation was indeed negligible and thus the reaction was kinetically controlled. Given that the reaction rates for the other CZC samples were found to be typically lower, in particular at temperatures below 573 K, the effect of intra particle transport phenomena on the kinetic parameters can be safely excluded.
The apparent activation energies were determined from the slopes of the Arrhenius plots, as expressed in Eq. 3.
A typical illustration of the Arrhenius parameters for the model reactions on CZC15 is displayed in Fig. 4. It may be readily observed from the slopes that the activation energies of SRM and RWGS are comparable and considerably lower than that of MD. The values of the activation energies for the SRM, RWGS and MD reactions, determined for all the CZC samples, are listed in Table 3.
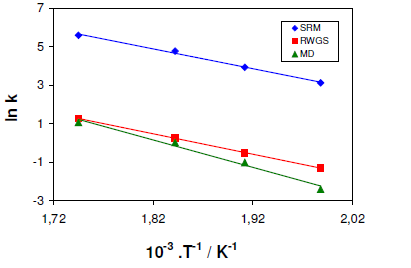
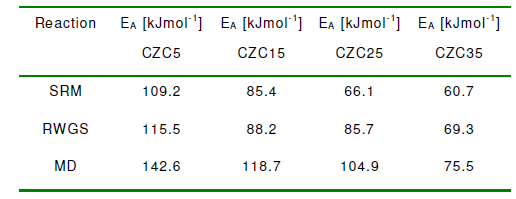
According to Table 2, there are no significant differences between the activation energies of the SRM and RWGS reactions can be observed, except for CZC25. On the other hand, the activation energies for MD proved to be noticeably higher than those of SRM and RWGS and therefore MD may be regarded as the rate-limiting step for carbon monoxide formation at short contact times. The results indicated that the overall reaction mechanism of MD was dependent on reaction temperature as it decreased dramatically at contact times exceeding 0.3-1 seconds.
On the other hand, the increase in carbon monoxide levels obtained at longer contact times can be caused by the predominance of RWGS reaction which occurs at considerably lower temperature. In addition, the activation energies estimated for the main reaction (SRM) were relatively closer for all the samples apart from CZC5 for which the highest value was obtained. For comparison purposes, we plotted the Arrhenius calculations in Figure 4 as shown above. Moreover, the CZC5 and CZC15, the apparent activation energies are in good agreement with those reported in the literature for Cu/ZnO/Al2O3 catalysts, whereas the values for CZC25 and CZC35 are considerably lower.
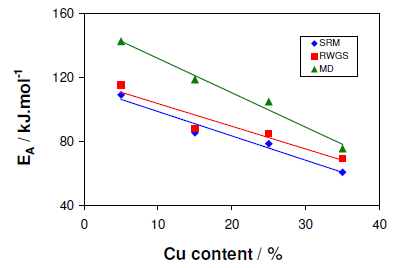
As shown in Figure 5, the model reactions of SRM, RWGS, and MD activation energies exhibited a linear correlation with the varying copper content. On increasing the amount of Cu, a systematic decrease can be observed for each reaction. The most pronounced decrease can be observed in the activation energy of MD, indicating that at lower Cu loading the contribution of MD to CO formation diminishes considerably. Yaakob et al. have reported a similar trend for the SRM reaction on two Cu/ZnO catalysts, although a potential mass transport limitation was not taken into account and thus their studies cannot be directly compared with the results presented here. However, in this work the effect of mass transport limitation on the kinetic studies those results in reduced activation energy can be excluded. Therefore, it is worthy to conclude that the variation of the Cu concentration in the CeO2/ZrO2 precursor material can give rise to the formation of CZC samples with significantly different catalytic behavior.
The chemical complexity in CZC samples remained the same in the whole procedure whereas the composition was significantly altered during preparation. These catalysts with vastly different active surfaces exhibit a catalytic behavior that is not simply correlated to the accessible surface area. Dispersion, Cu particle size, and Cu support interactions have a pronounced influence on the microstructure of the Cu particles and the effect of the microstructure on the active Cu surface. Eventually, both the Cu surface area and the micro-structural properties of the Cu particles need to be elucidated in order to fully understand the significantly different catalytic activities and the kinetics described above.
Conclusions
In this work novel Cu/ZrO2/CeO2 materials prepared by co-precipitation and polymer templating have been investigated as catalysts in the steam reforming of methanol. Catalytic measurements were performed under continuous operation in a fixed bed reactor at atmospheric pressure. Experiments performed indicated that an initial period of deactivation in CZC samples with Cu contents exceeding 5% were stabilized and therefore such samples are recommended for prolonged catalytic applications. An increase in the Cu content from 5 to 15% was found to improve the long-term stability and suppress the CO production considerably, whereas the effect of a further enhancement was less significant. With respect to a reduced formation of CO during SRM, the samples with increased Cu loadings proved to be more efficient catalysts. A kinetic analysis of the catalysts was undertaken as a result of insignificant effect of mass transport on the catalytic reaction. The reaction scheme suggested for the overall transformation included the SRM, MD and RWGS reactions. In addition, the experimental data obtained in the range 503-573 K could be well fitted by the kinetic model employed.
The optimal temperature range for SRM on the CZC catalysts was 523-543 K, for which high methanol conversions and low carbon dioxide levels were obtained. For the SRM and RWGS reactions, the values of the activation energies were comparable and considerably lower than that of MD. The activation energy of each individual reaction exhibited a systematic decrease with an increasing Cu content of the catalyst. Evidently, the variation in the Cu concentration of the precursor material during preparation altered the microstructure of the Cu particles and, thus the active Cu surface, which considerably affected the catalytic behavior of the CZC catalysts.
Catalyst preparation
Pellets of Zirconium oxynitride were isostatically pressed commercial Zirconia powder prepared at 1900°C in nitrogen atmosphere for 2 h in graphite heated resistance furnace. This resulted in a mixture of nitrogen-free monoclinic and beta-type Zirconium oxynitride phases. The resultant material was subsequently quenched from a vertical tube furnace from 1300°C (nitrogen atmosphere) in water. The X-ray analysis after quenching shows a reduced amount of monoclinic zirconia and metastable beta-phase of zirconium oxynitride (~Zr7O11N2). Samples containing 51 wt-% ZrO2 and 49 wt-% beta-phase were utilized during the analysis of the catalyst. Hot gas extraction of the as-prepared material yielded a total amount of nitrogen of 1.63 wt-% (theoretical 1.62 wt-%). A minor amount of nitrogen resulted after three month of ammonia decomposition.
In situ XRD
A STOE STADI P diffractometer equipped with a graphite heated resistance furnace was utilized for the high temperature X-ray diffraction experiments. The oxynitride powders were filled into capillaries under nitrogen and heated up at a rate of 20 Kmin-1. At a given temperature, diffraction data were collected for 15 minutes using a position sensitive detector after an annealing time of 15 minutes.
Catalyst testing
First the catalysis measurements of the decomposition of ammonia were performed at atmospheric pressure in a PFTR reactor (stainless steel) heated to the corresponding reaction temperature.
NH3 = 0.5 N2 + 1.5 H2 DH0 = +46.2 kJ/mol
A schematic drawing of the catalysis set-up used is depicted in Figure 1. The heat in the reactor was increased in steps of 20 K. This followed a stepwise increase in the conversion followed by slow decrease conversion of the steam. Steady state conversion was reached after about 10 min. The conversion data reported herein were determined after 20 minutes on stream at the corresponding temperature.
Schematic arrangement of experimental set up / P = pressure reducer, MFC = massflow controller, HE = heat exchanger, WF = wash flask, filled with H2SO4
The function of the mass flow controllers was to regulate the amount of ammonia and helium in the feed. Such a setting allowed use of different partial pressure of ammonia at different flow rates resulting in different contact times. In order to properly adjust regular flow rates, the massflow controller for ammonia required a special seal and a reliable pressure reducer of particular precision. The PFTR reactor was mounted into a heating block (brass, 80 mm in diameter, 150 mm length), equipped with six high temperature heating cartridges of 220 Watt and heated to the desired to the desired temperature.
The heating block prepared was used to accommodate three reactors. One of the reactors was loaded with glass beads which served as a blank reactor to determine the thermal and reactor contribution to the conversion of ammonia. In the second reactor which was filled with an iron oxide catalyst, acted as a reference catalyst for ammonia synthesis. The third reactor was filled in particles of Zirconium oxynitride catalyst. Glass beads were placed below and above the catalyst bed to ensure that the feed gases and the catalyst powder possess the same preset temperature in the reaction zone.
To ensure that the product streams of different experiments are at the same temperature before they reach the ammonia sensor, the outlet gases were cooled to -10°C. Afterwards the outlet gases were washed with sulfurid acid. 4-(Dimethylamino)-benzaldehyde was added to test for hydrazine in the reaction product. Even after one month of time on stream, no hydrazine was detectable. During this time 2.25 mol of ammonia were passed through the reactor. The detection limit for the hydrazine assay is 0.0005 mg/L, which means formation of <0.4 ppm of hydranzine. Result of catalyst testing and catalyst characterization
Zirconium oxynitrides are nitrogen conducting that have displayed a promising novel ammonia decomposition catalysts that completely avoid the presence of neighboring reduced metal sites. This effect is due to the combination of an appropriate intrinsic reactivity towards nitrogen and high resistance towards reduction which makes the materials promising for the production of hydrogen. In the following section we describe first catalysis studies linking the catalytic behavior in the decomposition of ammonia to the inception of nitrogen mobility using zirconium oxynitrides catalyst. Figure 3 shows an X-ray analysis of the as-prepared material of monoclinic zirconia and the metastable beta-phase zirconium oxynitride. This material was used for catalytic activity testing and is referred to as ZrON in this work. The conversion of ammonia to nitrogen and hydrogen as a function of temperature on a conventional catalyst of Fe3O4, the ZrON catalyst (heating and cooling) and the blank reactor (glass beads) is depicted in Figure 4.
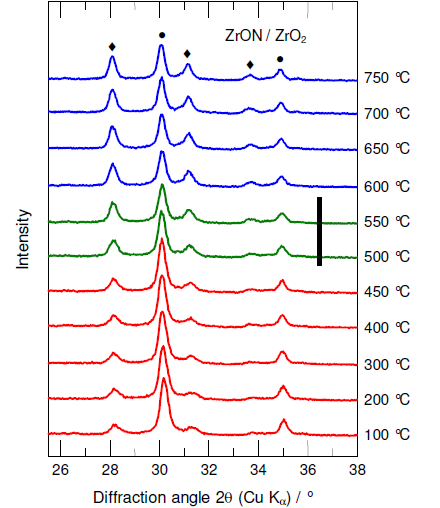
As shown in Fig. 4, iron oxide had an increased onset activity in the decomposition of ammonia at ~340°C. However, no detectable activity was seen for the as-prepared ZrON in temperatures below 550°C. Conversely, a jump of temperature was clearly exhibited in the ammonia decomposition in temperature above 550°C. This was followed by cooling the catalyst down to ambient temperature. However, cooling the catalyst down led to subsequent decrease in version of ammonia to its constituents.
When compared to the conventional iron oxide catalyst, the abrupt onset activity of ZrON at ~550°C does not match the known increase in activity with increasing reaction temperature. This was seen as a corresponding behavior of endothermic nature of ammonia decomposition reaction that results in an ignition behavior of the reactor. Therefore, sudden bulk structural changes have to be assumed as origin of the catalytic activity observed. As known from our high-temperature XRD work, reheating the as-prepared metastable mixture of nitrogen-poor beta-phase (Zr7O11N2) and nitrogen-free monoclinic ZrO2 in nitrogen results in a further demixing to the nitrogen-rich phase (Zr7O9.5N3) and an increased amount of m-ZrO2 at 550°C (Figure 3).
The structures of the ß’- and ß’’-phases can be described as anion-deficient fluorite types with ordered anion vacancies, whereas the ß’-phase is strongly disordered. The results showed that the b” phase ZrON contains a larger amount of nitrogen ions in the lattice than the b´phase, which is also accompanied by an increased amount of vacancies in the oxygen sublattice. The phase demixing required an increased mobility of nitrogen ions in the ZrON structure. Apparently, this enabled considerable migration of nitrogen ions and the formation of b”
ZrON and ZrO2. Furthermore, the change of the b” to b’ ZrON phase was a good indicator for the onset of nitrogen ion in the as-prepared ZrON catalyst. As depicted in Figure 3 the sudden onset of catalytic activity in ammonia decomposition on ZrON is clear and this corresponds to the phase change from the initial b’ phase to b” ZrON catalyst. The the b´ phase exhibits only negligible activity in ammonia decomposition while the b´´ ZrON phase possesses a considerably activity similar to that of a conventional iron catalyst. This led to us envisaging that the b” phase exhibits an increased nitrogen mobility compared to the b’ phase, in addition to the increased amount of nitrogen in the b” phase. However, the reduced mobility and amount of nitrogen in the initial b’ phase is not sufficiently enough fact to merit a detectable catalytic activity.
In a recent study carried out on ZrON, the X-ray diffraction showed that the the b´´ phase persists upon cooling and does not change back to b´. Such a metastability of the b´´ phase is reflected in the conversion of ammonia during the period of cooling process. Importantly, even below the phase demixing temperature, the increased amount of nitrogen and its mobility in the b´´ ZrON phase resulted in an improved activity compared to the b´ phase. Furthermore, the ammonia conversion on b´´ ZrON exhibited a more regular behavior as a function of the decreasing temperature. The effect of subsequent increase in temperature on the ZrON catalyst on ammonia resulted in step size increase in the activity of the catalyst that coincided with the cooling curve of the first temperature cycle.
The activation energy of ammonia decomposition on ZrON catalysts was determined as a function of temperature. This entailed varying the partial pressure of ammonia in the feed followed by determining the conversion rate. Figure 4 depicts the conversion and it can be concluded that the ZrON catalyst is apparently independent of the ammonia partial pressure. Additionally, the assumption that ZrON catalyst was not saturated under the applied conditions was proved correct as the reaction was a first order reaction.
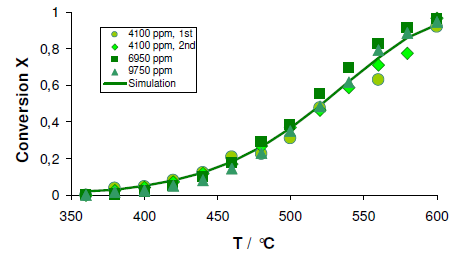
The activation energy was determined by fitting the equation below to the conversion data as a function of temperature.
Activation energy of 106 ±10 kJ/mol-1 was obtained at temperatures between 400 – 550 °C. This activation energies are in good agreement with other activation energies assumed for ion conductivity in zirconium oxynitride based materials. Further calculations were done for the uncatalysed reaction from the conversion data and an activation energy of 70 ± 10 kJ/mol-1 resulted. The absence of detectable hydrazine is an indicative of a mechanism of ammonia decomposition on ZrON that varies from that of conventional reduced metal catalysts. This confirms the sudden onset of the catalytic behavior and nitrogen mobility in the new mechanistic concept for this new class of materials.
Further analysis done using XPS revealed an increasing amount of nitrogen at the surface of the ZrON after heating to temperatures of 600°C. The results are shown in Figure 5 and Table 1 below.
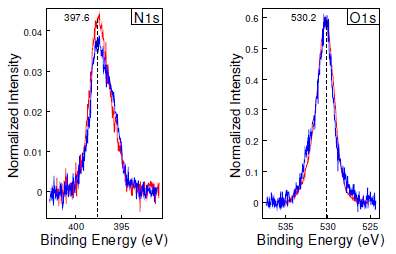
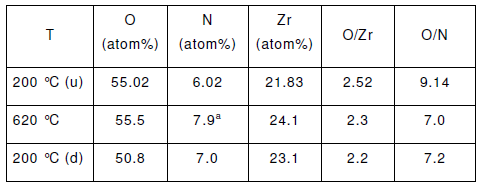
Measured at 600
A schematic diagram illustrates the how the defects sites of ZrON constitute the active sites for the adsorption of ammonia. Dinitrogen molecule is subsequently formed with a nitrogen atom from the lattice of ZrON catalyst. The final step involves the replenishing of the vacant generated vacancy by nitrogen diffusion from the bulk or ammonia from the gas phase as shown below in Figure 5.
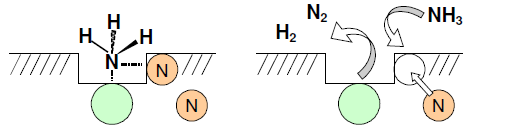
Conclusion
In conclusion, the Zirconium oxynitride studied in this work showed a considerable remarkable catalytic activity in the decomposition of ammonia. On the other hand, conventional metal containing catalysts exhibited a remarkable hydrazine formation during extended time on the stream. ZrON gave no detectable amounts of hydrazine and therefore is considered in the production of energy. In addition, the observed sudden increase in activity at ~550°C coincides with the onset of nitrogen ion conductivity in the material and the phase change from the initial ß’ phase to the nitrogen rich b“ ZrON phase.
On the other hand, addition of hydrogen has a negative effect of inhibiting the reaction whereas nitrogen does not influence the conversion. Moreover, apparent activation energy of 110 ± 10 kJ/mol agrees well with the activation energy of ion mobility in zirconia based materials. The dramatic change in activity is also correlated to a rapid change in the electronic structure of the surface that accompanies the formation of the more active b” ZrON phase. The results presented show for the first time a direct correlation between the onset of ion conductivity as a bulk property, a modified electronic structure of the surface, and the catalytic performance of a heterogeneous catalyst.
Recommendation
The most appropriate system for on board hydrogen production is the steam reforming methanol due its advantages regarding to the high hydrogen content of methanol, the absence of C-C bond in the reactants as well as the moderate reaction temperature. Cu-based catalysts are widely applicable and are known as active and quite selective candidate. In this research work, steam reforming methanol was investigated over Cu/ZrO2/CeO2 (CZC) catalysts prepared via a novel synthetic method based on co-precipitation and polymer templating. The catalysts investigated in this work are very active and requires no activation period and because of its porosity there is no significant diffusion limitation. Accordingly, the variation of Cu loading resulted in an increased Cu crystallite size and a reduction in surface area of the active particles was observed. In addition, samples with Cu contents higher than 5% are the most applicable due to their long term stabilities and low CO levels during a continuous operation.
The temperature for methanol conversion should range 523-543 as this gives highest amount of hydrogen. Steam reforming of methanol is an endotherm process and the hydrogen production by SRM over copper based catalysts has still a problem in respect of the undesirable production of carbon monoxide. These two aspects need to be solved and must be taken into account if we want to produce hydrogen on board for mobile application. One will be a process to reduce carbon monoxide content to tolerable limits. However, there is no chemical solution of CO minimization and an effort in this direction is recommended for further work. A new reactor and platinum electrodes of the fuel cells can be investigated. The second strategy to minimize the production of CO is finding another hydrogen carrier with no carbon content such as ammonia. Although ammonia is widely found and cheap, and no carbon dioxide is produced, sufficient amount of hydrazine is produced rendering it non-friendly to environment. Our work on hydrazine-free catalysts is a promising perspective in ammonia decomposition.
References
Balat, M., Microbial Fuel Cells as an Alternative Energy Option. Energy Sources Part A-Recovery Util. Environ. Eff. 32 (1), 26-35.
Richter, B.; Goldston, D.; Crabtree, G.; Glicksman, L.; Goldstein, D.; Greene, D.; Kammen, D.; Levine, M.; Lubell, M.; Savitz, M.; Sperling, D.; Schlachter, F.; Scofield, J.; Dawson, J., How America can look within to achieve energy security and reduce global warming. Rev. Mod. Phys. 2008, 80 (4), S1-S107.
Naik, S. N.; Goud, V. V.; Rout, P. K.; Dalai, A. K., Production of first and second generation biofuels: A comprehensive review. Renew. Sust. Energ. Rev. 14 (2), 578-597.
Budzianowski, W. M., Role of catalytic technologies in combustion of gaseous fuels. Rynek Energii 2009, (3), 59-63.
Tanksale, A.; Beltramini, J. N.; Lu, G. M., A review of catalytic hydrogen production processes from biomass. Renew. Sust. Energ. Rev. 14 (1), 166-182.
Yu, S.; Hong, D.; Lee, Y.; Lee, S.; Ahn, K., Development of a catalytic combustor for a stationary fuel cell power generation system. Renew. Energy 35 (5), 1083-1090.
Ozkara-Aydinoglu, S.; Ozensoy, E.; Aksoylu, A. E., The effect of impregnation strategy on methane dry reforming activity of Ce promoted Pt/ZrO2. Int. J. Hydrog. Energy 2009, 34 (24), 9711-9722.
Fan, M. S.; Abdullah, A. Z.; Bhatia, S., Catalytic Technology for Carbon Dioxide Reforming of Methane to Synthesis Gas. ChemCatChem 2009, 1 (2), 192-208.
Cai, X. L.; Cai, Y. X.; Lin, W. M., Autothermal reforming of methane over Ni catalysts supported over ZrO2-CeO2-Al2O3. J. Nat. Gas Chem. 2008, 17 (2), 201-207.
Eom, K.; Kim, M.; Kim, R.; Nam, D.; Kwon, H., Characterization of hydrogen generation for fuel cells via borane hydrolysis using an electroless-deposited Co-P/Ni foam catalyst. J. Power Sources 195 (9), 2830-2834.
Srisiriwat, N.; Therdthianwong, S.; Therdthianwong, A., Oxidative steam reforming of ethanol over Ni/Al2O3 catalysts promoted by CeO2, ZrO2 and CeO2-ZrO2. Int. J. Hydrog. Energy 2009, 34 (5), 2224-2234.
Fumoto, E.; Matsumura, A.; Sato, S.; Takanohashi, T., Kinetic Model for Catalytic Cracking of Heavy Oil with a Zirconia-Alumina-Iron Oxide Catalyst in a Steam Atmosphere. Energy Fuels 2009, 23, 5308-5311.
Yin, F. X.; Takanabe, K.; Kubota, J.; Domen, K., Polymerized Complex Synthesis of Niobium- and Zirconium-Based Electrocatalysts for PEFC Cathodes. Journal of the Electrochemical Society 2010, 157 (2), B240-B244.
Wu, C. F.; Williams, P. T., Ni/CeO2/ZSM-5 catalysts for the production of hydrogen from the pyrolysis-gasification of polypropylene. Int. J. Hydrog. Energy 2009, 34 (15), 6242-6252.
Gates, B. C.; Huber, G. W.; Marshall, C. L.; Ross, P. N.; Siirola, J.; Wang, Y., Catalysts tor emerging energy applications. MRS Bull. 2008, 33 (4), 429-435.
Rizzo, A.; Signore, M. A.; Mirenghi, L.; Piscopiello, E.; Tapfer, L., Physical properties evolution of sputtered zirconium oxynitride films: effects of the growth temperature. J. Phys. D-Appl. Phys. 2009, 42 (23), 8.
ENERGY FUTURE: Think Efficiency. Rev. Mod. Phys. 2008, 80 (4), S6-S103.