Abstract
Groundwater quality in many countries has special significance and needs great attention of all concerned since it is the major alternate source of domestic, industrial and drinking water supply. The present study monitors the ground water quality, relates it to the land use / land cover and maps such quality using Remote sensing and GIS techniques for a part of Western Cape, South Africa metropolis. Thematic maps for the study are prepared by visual interpretation of SOI topo-sheets and linearly enhanced fused data of IRS-ID PAN and LISS-III imagery on 1:50,000 scale using AutoCAD and ARC/INFO software.
Physico-chemical analysis data of the groundwater samples collected at predetermined locations forms the attribute database for the study, based on which, spatial distribution maps of major water quality parameters are prepared using curve fitting method in Arc View GIS software. Water Quality Index (WQI) was then calculated to find the suitability of water for drinking purpose. The overall view of the water quality index of the present study area revealed that most of the study area with > 50 standard rating of water quality index exhibited poor, very poor and unfit water quality except in places like Banjara Hills, Erragadda and Tolichowki. Appropriate methods for improving the water quality in affected areas have been suggested.
Introduction
The urban environment quality is fading day by day with the largest cities reaching saturation points and unable to cope with the increasing pressure on their infrastructure. Rooiberg Winery is situated on the scenic R60 route that connects the Western Cape (South Africa) towns of Robertson and Worcester. North latitude is facing a rapid change in the environmental quality. Rapid urbanization brings with it many problems as it places huge demands on land, water, housing, transport, health, education etc. Environmental pollution has reached alarming levels in the last 5-6 years mainly due to industries and automobiles.
The city witnessed an increase in population from 0.448 million in 1901 – 1.429 million in 1961, between 1981 and 1991 the population went upto 4.34 million and the growth rate so far is 67.04%. As per the population estimates,This rising population density will continue to have an impact on the quality and quantity of local water resources.
Fresh water being one of the basic necessities for sustenance of life, the human race through the ages has striven to locate and develop it. Water, a vital source of life in its natural state is free from pollution but when man tampers the water body it loses its natural conditions. Ground water has become an essential resource over the past few decades due to the increase in its usage for drinking, irrigation and industrial uses etc. The quality of ground water is equally important as that of quantity.
Remote sensing and GIS are effective tools for water quality mapping and land cover mapping essential for monitoring, modelling and environmental change detection. GIS can be a powerful tool for developing solutions for water resources problems for assessing water quality, determining water availability, preventing flooding, understanding the natural environment, and managing water resources on a local or regional scale. Keeping this in view, we have Integrated Remote Sensing, GIS and field studies for the evaluation of the impacts of land use changes on the ground water quality of zone-V under MCH.
So How Much Ground-Water Do We Have?
In total, some 235,5 billion cubic metres of groundwater may be stored in aquifers in South Africa. Of course, not all of it is usable and can be abstracted. There are many limitations to the possible abstraction of groundwater for use, for example, restrictions to ensure enough water for the environment (the Ecological Reserve), and restrictions on the maximum level drawdown in dolomitic aquifers due to the hazard of sinkhole formation or avoiding intrusion of saline water.
The groundwater resource potential is the maximum volume (m3) of groundwater that can be abstracted per unit area per annum without causing any long-term ‘mining’ of the aquifer system (i.e. without continued long-term declining water levels). It is not equivalent to the sustainable or optimal yield of the system, which normally takes into account issues such as intrusion of poor quality water, practical and cost issues relating to extracting the water and so forth. The average groundwater resource potential of aquifers in South Africa is estimated under normal rainfall conditions at 49 billion m3/a, which decreases to 42 billion m3/a during a drought.
For general planning purposes, it is recommended that the average utilisable groundwater exploitation potential volume be adopted. It is interesting to note that only about 20% of this volume is currently being abstracted on an annual basis
Groundwater Resource Potential
The Groundwater Resource Potential (GRP) is defined as the maximum volume (m3) of groundwater that can be abstracted per unit area per annum without causing any long-term ‘mining’ of the aquifer system (i.e. without continued long-term declining water levels). The GRP is based purely on physical inputs / outputs and aquifer storage. It is therefore not equivalent to the ‘sustainable’ or ‘optimal’ yield of the system, which normally takes into account factors such as intrusion of poor quality water, variable aquifer permeability, practical and cost issues relating to extracting the water etc.
Two basic algorithms have been developed to determine the GRP based on the
- average or steady-state (AGRP) and
- dynamic or transient-state aquifer conditions.
The steady-state Groundwater Resource Potential dataset is similar to DWAF’s Harvest Potential map in that they both provide estimates of the maximum volumes of groundwater that are potentially available for abstraction on a sustainable basis, and only take into consideration the volumes of water held in aquifer storage and the recharge from rainfall. The feasibility of abstracting this water is limited by many factors due mainly to the physical attributes of a particular aquifer system, economic and/or environmental considerations.
One of the most important of these is the inability to establish a network of suitably spaced production boreholes to ‘capture’ all the available water in an aquifer system or on a more regional scale (Water Systems Management, 2001). The factors limiting the ability to develop such a network of production boreholes, include, inter alia, the low permeability or transmissivity of certain aquifer units, accessibility of terrain to drilling rigs, unknown aquifer boundary conditions.
Groundwater Exploitation Potential
In order to account for the pumping limitations discussed above, the GRA2 project made use of Haupt’s (2001) concept of an ‘Exploitability Factor’ or EF and Vegter’s (1995) national ‘Borehole Prospects’ coverage to generate a 1x1km EF grid for the country. Vegter stated that the prospects of obtaining a groundwater supply from a particular lithological unit may be judged by analysis of the yield distribution of an adequate number of randomly spaced boreholes drilled into this unit. He classified the lithostratigraphic units of the country into 16 water-bearing categories and analysed the yield information from 120,000 boreholes obtained from DWAF’s NGDB. The Borehole Prospects coverage is therefore an indication of the extent to which various lithological units are able to act as aquifers.
The EF factor was applied to the AGRP grid to produce the so-called ‘Average Groundwater Exploitation Potential’ or AGEP coverage. The total AGEP of aquifers in South Africa is estimated at 19,073 Mm3/a, which declines to 16,253 Mm3/a during a drought. It is likely that, with an adequate and even distribution of production boreholes in accessible portions of most catchments or aquifer systems, these volumes of groundwater may be annually abstracted on a sustainable basis.
Groundwater quality is one of the main factors restricting the development of available groundwater resources. Although there are numerous problems associated with groundwater quality, some of which are relatively easily remediated, high concentration of total dissolved solids, nitrates and fluoride are considered to be the most common and serious problems associated with water quality on a regional scale.
Potable Groundwater Exploitation Potential
The Potable Groundwater Exploitation Potential (PGEP) of aquifers in South Africa is estimated at 14,802 Mm3/a, which declines to 12,626 Mm3/a during a drought. Nationally, this represents almost a 30% reduction in the annual volumes of available groundwater for domestic supply due to water quality constraints.
The volume of water that may be abstracted from a groundwater resource may ultimately be limited by anthropogenic, ecological and/or legislative considerations, which ultimately is a management decision that will reduce the total volume of groundwater available for development – referred in the GRA2 project as the Utilisable Groundwater Exploitation Potential (UGEP). This includes the important legislative restriction imposed on the volumes of groundwater available for utilisation by the requirements of the ‘Groundwater Component’ of the Reserve as stipulated in the South African National Water Act of 1998. Other aspects such as protection against the hazards of saline intrusion or sinkhole formation, conserving important groundwater dependant ecosystems, maintaining base flow to rivers etc. can all be factored in using this approach.
The Utilisable Groundwater Exploitation Potential (UGEP) under normal rainfall conditions and under drought conditions is estimated at 10,353 and 7,536 Mm3/a, respectively. The UGEP represents a management restriction on the volumes that may be abstracted based on the defined ‘maximum allowable water level drawdown’ and therefore it is always less than or equal the AGEP. It is likely that, with an adequate and even distribution of production boreholes in accessible portions of most catchments or aquifer systems, these volumes of groundwater may be annually abstracted on a sustainable basis.
Study Area
The mapping area lies between latitudes 33° 40′ 45″ – 33° 30′ 12″ N and longitudes 21° 22′ 24″ – 21° 23′ 05″ E and covers an area approximately 766 km2. Ladismith is located in the Western cape region about 400 km east of Cape Town. The Rooiberg Mountain range sits in the Klein Karoo region Between the Groot Swartberg and the Lange Berg. The area was chosen because of its environment and its dependency on groundwater, especially by the many farms located within the Rooiberg catchments. The Rooiberg Mountain range is part of the centrepiece of the Cape Fold Belt (CFB) and represents an anticlinal structure. Geologically the mapping area comprises of the harder Table Mountain Sandstone (TMS) and the softer shale’s of the Bokkeveld groups.
Table 1: Important features relating to the study area.
Methodology
In this present study, the methods used follows a combination of techniques already used in previous studies of a similar nature. Various datasets and maps (Table 2) of different scales were used. In order to demarcate groundwater potential zones, thematic maps were created either from remote sensed or from conventional field data. All the data that wasn’t in a digital format was digitized and spatial database was developed. Those thematic maps created from remote sensing included:
- geology,
- Landforms and
- Lineaments.
The remote sensing data was acquired from the NASA Multispectural Scanner Landsat 2000 Geocover collection (spectral range 0.450 – 2.35 µm), path 173 / row 83. The secondary base maps of
- Drainage density,
- Slope and
- elevation were prepared from 1:50 000 scale topographic maps of the Ladismith region (3322CB).
Digital topographic maps of the area were acquired from the Chief Directive Survey and Mappings based in Cape Town, South Africa. Other supportive data such as boreholes and spring locations (GPS) were collected out in the field. Other miscellaneous data like borehole levels and rainfall data (appendices) were collected from the local farmers. All suitable field data was digitized and included during the preparation of the thematic maps.
In order to create the spatial database, the ESRI ArcGIS (ArcMap 9.2, ArcCatalog and ArcScene) software was utilised during the integrated analysis. ArcCatalog was used extensively in building and managing the spatial database. ArcMap 9.2 with the ‘Spatial Analyst’ extension was used for creation and analysis of the thematic maps. ArcSecne was used to build a 3D representation of the study area in conjunction with the topography. The development of the thematic maps follows the similar methods used by Kumar et al. (2007), Krishnamurthy et al. (1996) and Saraf et al. (2000), all the base maps were geo-referenced to a common reference point.
This was done by using the 1:50 000 topographic map of the Ladismith region, using ground control points common to all maps (ESRI 2008). Thematic maps such as geology, lineaments and landforms were derived by visual interpretation of the Landsat 2000 Geocover. All the thematic maps were digitized in the vector format whereby the values of the polygons were edited and labelled. After this a multi-criteria evaluation technique (Chi & Lee, 1994) was used to assign weightings and scores to the various thematic map themes depending on the features influence on groundwater occurrence (Table 3).
The suitable weights were determined by the various groundwater-controlling parameters (Table 4). For example, in this study geology plays a vital role in groundwater storage followed by landforms, lineaments, drainage density, slope and then elevation. The total weight of 100 was divided between the themes, whereby geology was assigned a maximum of 45 and a minimum of 5 was assigned to elevation and so on (See map algebra). All the themes and features were then converted into raster format using the ‘Spatial Analyst’ extension of ArcMap 9.2. The scores of the individual features were entered in the value field and all the themes were overlaid two at time by using the ‘Weighted Sum’ tool in ArcMap 9.2. The final groundwater potential zone map was classified from very good to very poor. The location of boreholes and springs were used to correlate this output. The steps for the methodology are shown in flowchart (figure 3).
Groundwater Potential Map (GPM) = (Geology) x 0.45 + (Landforms) x 0.25 + (Lineaments) x 0.10 + (Drainage De Methodology.
Table 2: Various datasets used in the study.
Geology
In the study area, the Table Mountain and Bokkeveld groups underlie most of the Klein Karoo. The majority of the rocks that form the Table Mountain Group (TMG) are made up of medium-grained quartzites and sandstones. The TMG comprises of the Upper and Lower Table Mountain Sandstones and that of the Pakhuis formations. The Pakhuis formation is situated between the lower and upper TMS and comprises mainly of shales and diamictites. The Pakhuis formation is less competent than the TMS sandstone and quartzite and so is more susceptible to weathering.
In contrast to the TMS, the rocks of the Bokkeveld are also less competent than the TMS. They consist mainly of sandstone, shales and massive quartzites that make up most of the ridges seen in remote sensing. On the mapping area the Bokkeveld group is divided into eight subdivisions, field information and the geological map were used to distinguish the different rock units from each other. Very few of the formations are made up of one type of rock, the sandstones, shales and quartzites appear in many formations but in different orders, ratio and relation.
The different geologic units were outlined from the remote sensing images by the distinct characteristics. On the False Colour Composite (FCC) image, the TMG was identified on the image by areas of reddish-brown to blue colour/tone with medium to fine texture, very distinct on the northern slope of the Rooiberg. The Bokkeveld formations were demarcated by the bluish-brown to green in colour and tone. The presence of shale shows up to medium to coarse in texture, owing to weathering and surfaces of the shale composition. Alluvium was clearly outline by the reddish colour from the vegetation and the light-yellow colour/tone of the sediments. Bare surfaces such as roads and low lying areas appeared white on the FCC image.
Borehole information
Most of the previous work has been in the western and central parts of the Karoo Basin, which has only a small fraction of the population of the eastern part. Although thousands of boreholes have been drilled in the eastern parts, the data, such as borehole depths, depths of water strikes, drilling yields, etc, does not exist in a useful and readily available format. In order to develop reasonable conceptual models describing the occurrence and flow dynamics of groundwater in the Eastern Karoo Basin, this data will need to be captured in a manner that enables spatial and statistical analysis.
Borehole and groundwater information (data capture and analysis)
The collection of appropriate data is essential not only for research purposes but also for the day-today aquifer management, as well as for developing long-term water resource planning strategies. In relation to groundwater occurrence, borehole information including depths, water strikes, drilling yields, geology and water quality needs to be captured in a spatially and statistically useable format.
This will require updating and integrating various data sets, including the older National Groundwater Database (NGDB) and its replacement the National Groundwater Archive (NGA), as well as local initiatives such as the Eastern Cape Province’s Groundwater Information (GRIP) Project. In relation to sustainability, water level, abstraction and water quality time-series data needs to collected. This information is crucial if aquifers are to be developed to their maximum capacity and managed in a sustainable manner. The following types of questions need to be addressed: What data should be collected, and how often? How should the data be assessed and presented in order to
Groundwater Research Needs i n the Eastern Karoo Bas in of South Africa
May 2006 provide useful information for Water Service Authorities? What other support in terms of data collection and analysis can groundwater professionals provide to the Water Service Authorities?
Landforms
Effectively, landforms act as surficial indicators to groundwater potential. Each landform is different physiographically and the genesis and processes of different geomorphic units is determined by the underlying lithology, slope and the type of drainage pattern that exists (Saraf, 1999). Again the recognition of the various landforms were outlined according to tone, texture, size and shape. In total seven geomorphological units were identified. In the centre of the mapping area is the eastern section of the Rooiberg range with steep rising slopes that represents an anticlinal structure caused by compression during the CFB orogeny. To the south and north are the undulating hills of the Bokkeveld.
The landforms that were mapped from the remote sensing images: Linear Ridges, Denudo-Structural Hills, Structural Hills, Residual Hills, Residual Mount Complexes, Pediplains, Valley Fill and Alluvial Fill.
- Linear Ridges: Very few linear ridges are found in the mapping area, those that fall within the mapping area were located in the upper TMS of the Rooiberg. Its linear feature, steep slopes and reddish-brown to blue in colour and tone identified this landform on the FCC. Anticlinal and synclinal folding shows up as long linear parallel ridges and valleys.
- Denudo-Structural Hills: A main linear ridge that runs in an E-W trend separates the denudation-structural hills, which is present only in Rooiberg itself. These features can be separated into a southern complex and a northern complex. These were delineated by the homogenous structural shape, ruggedness and reddish-brown to bluish-purple in colour/tone on the FCC. Other identifiable features from this landform are its medium to fine texture and areal extent in terms of its drainage density.
- Structural Hills: Structural hills at the base of the Rooiberg to north and south are made up Boplaas Bokkeveld and Witterberg formations. The section of hills in the northwest corner of the mapping area consists of Wagen Drift Witterberg. Since the major rock types of these subdivision consists of sandstones, quartzites and siltstones with thin interlayers of shale, on the FCC the colour/tone of these features show up as reddish-brown. Theses hills are also identified by the medium texture with bedding traces, saddles strike ridges and structurally controlled drainage.
- Residual Hills: These are found mostly in the southern part of the mapping area. Delineated by their detached, isolated nature with gentle slopes that show little folding. Residual hills are features from the end process of pediplination; they represent the reduction of the original mountain masses in the form of scattered hillocks on the Pediplains (Thornbury, 1990). Their structural shape, coarse to medium texture and dark red-green in colour and tone on the FCC, determined the identification of residual hills.
- Residual Mount Complexes: These mount complexes are formed by the prolonged erosion and weathering of pre-existing surfaces and original complex tectonic mountains. Collectively they form a complex of hills whereby the lithology varies in accordance with the competency of the rock. These complexes are found to the northwest and south of the mapping area. They were identified by variation of colour/tone from bluish-green to red. Again the textured also varied, those complexes in the south were found to be coarser owing to the lack of vegetation, whereas those complexes in the northwest were coarse to medium indicating more vegetation coverage. Structure and shape show the mount complexes not to have a structurally controlled drainage pattern although faulting is present.
- Pediplains: These features are gently undulating landscapes that sit between isolated residual hills and complexes. They vary in material and thickness covered by soil that originates from weathered material from the surrounding uplands. On the FCC the colour and tone of the Pediplains varied from white to greenish-blue and even from reddish-black owing to the soil cover. Again the texture is seen to be coarse-medium to even fine in the north section of the mapping area. These plains are situated mostly in lower-lying areas of the Bokkeveld, below the TMS.
- Valley Fill: This feature is characterised by unconsolidated alluvial and or colluvial material. Mostly situated within the Rooiberg itself on both the northern and southern flanks of the range. These areas are characterised by their broad to narrow structural drainage patterns. The dense vegetation that shows up to be bright red on the FCC with a coarse texture easily distinguishes the identification of valleys.
- Alluvial Fill: Characterised by meandering river channels that are perennial in the mapping area. These are found in the northwest/east corner and directly south of the Rooiberg in the mapping area. The unit shows up on the FCC with a smooth greyish-white texture from the sediments and also red in colour/tone from vegetation.
Lineaments
Lineaments act as pathways for ground water movement and are very important hydrogeologically (Kumar et al. 2007). They appear as large-scale linear features that are generally manifested by topography. The geological structure normally encountered in hardrock areas of places such as Africa and India is granite or granite gneiss overlain by a variable thickness of weathered material (Barker, 2001). Area and zones of hard rock terrain that contain lineaments, represents faulting and fracturing that results in increased secondary porosity and permeability. Hence this is why the majority of the large-scale lineaments are found within the TMS due to intense deformation. They pose as important structures for governing recharge, migration, and discharge of groundwater (Fetter, 1994).
A total of 86 major lineaments were identified in the mapping area with lengths varying from hundreds of meters to tens of kilometres. The lineaments can be broken up into those in north of the Bokkeveld (total = 23, NW-SE trend), that of Rooiberg TMG (total = 49, EW, NW-SE and NE-SW trend) and the rest in the south of the Bokkeveld (total = 14, NW-SE trend). By observing the lineament directions and lineaments intersections, it is evident that the structural patterns of the landforms are directly influenced.
Drainage Density
Significance of drainage pattern
The drainage system, which develops in an area, is strictly dependent on the slope, the nature and attitude of bedrock and on the regional and local fracture pattern. Drainage, which is easily visible on remote sensing imagery, therefore reflects to varying degrees the lithology and structure of a given area and can be of great value for groundwater resources evaluation. Drainage is studied according to its pattern type and its texture (or density of dissection) (Way, 1973).
Whilst the first parameter is associated to the nature and structure of the substratum, the second is related to rock/soil permeability (and, thus, also to rock type). Actually, the less a rock is permeable, the less the infiltration of rainfall, which conversely tends to be concentrated in surface runoff. This gives origin to a well-developed and fine drainage system. On the other hand, in karst regions, where the underground circulation of water is much more developed than the surficial one, drainage is less developed or missing altogether.
Six basic types of drainage patterns were identified, namely: dendritic, trellis, parallel, radial, anular and rectangular. Their features and occurrence are as follows (Way, 1973):
- In the dendritic pattern, a tree-like branching of tributaries join the mainstream at acute angles. Usually this pattern occurs in homogeneous rocks such as soft sedimentary or volcanic tuffs.
- Trellis is a modification of dendritic, with parallel tributaries converging at right angles. It is indicative of bedrock structure rather than material of bedrock. It can be associated to tilted or interbedded sedimentary rocks, where the main channels follow the strike of beds.
- In the parallel pattern, major tributaries are parallel to major streams and join them at approximately the same angle. It can occur in homogeneous, gentle and uniformly sloping surfaces whose main streams may indicate a fault or fracture zone. Common in pediment zones.
- The radial pattern is a circular network of approximately parallel channels flowing away from a central high point. It usually occurs in volcanoes or domelike structures characterized by resistant bedrock.
- Anular pattern is a concentric network of channels flowing down and around a central high point. This pattern is usually controlled by layered, jointed and fractured bedrock, in granitic or sedimentary domes.
- The rectangular is a modification of the dendritic pattern, with tributaries joining mainstream at right angles, forming rectangular shapes. It is controlled by bedrock jointing, foliation and fracturing, indicative of slate, schist, gneiss and resistant sandstone.
Further modifications of the six basic schemes give origin to more than 20 other patterns that cover almost all the possible existing cases.
In addition to the pattern characterization, drainage can also be described in terms of texture or density of dissection. On this basis, three types can be identified: 1) fine, which is indicative of high levels of runoff, suggesting impervious bedrock and/or fine textured soils scarcely permeable; 2) medium, which can be related to a medium runoff and mixed lithology, and 3) coarse, which indicates little runoff and consequently resistant, permeable bedrock and coarse, permeable soil materials.
Data interpretation
Data Used
Different data products required for the study include the 56K/7 and 56K/11 toposheets which are obtained from Survey of India (1:50,000) and fused data of IRS – 1D
PAN and LISS-III satellite imagery of path 100 and row 60 from National Remote Sensing Agency (NRSA), south Africa.
Database Creation
IRS-ID PAN and LISS-III satellite imageries are geo-referenced using the ground control points with SOI toposheets as a reference and further merged to obtain a fused, high resolution (5.8m of PAN) and coloured (R,G,B bands of LISS-III) output in EASI/PACE v6.3 Image processing software. The study area is then delineated from the fused data based on the latitude and longitude values and a final hard copy output prepared which is further interpreted visually for the generation of thematic maps.
These thematic maps (Raster data) are converted to vector format by scanning using an A0-Flatbed DeskJet scanner and digitized in AUTOCAD 2000. The map is further edited in ARC/INFO v3.5.1 and final hardcopy output is prepared using ARC/VIEW v3.1 GIS software. The methodology adopted for creation of database is given in
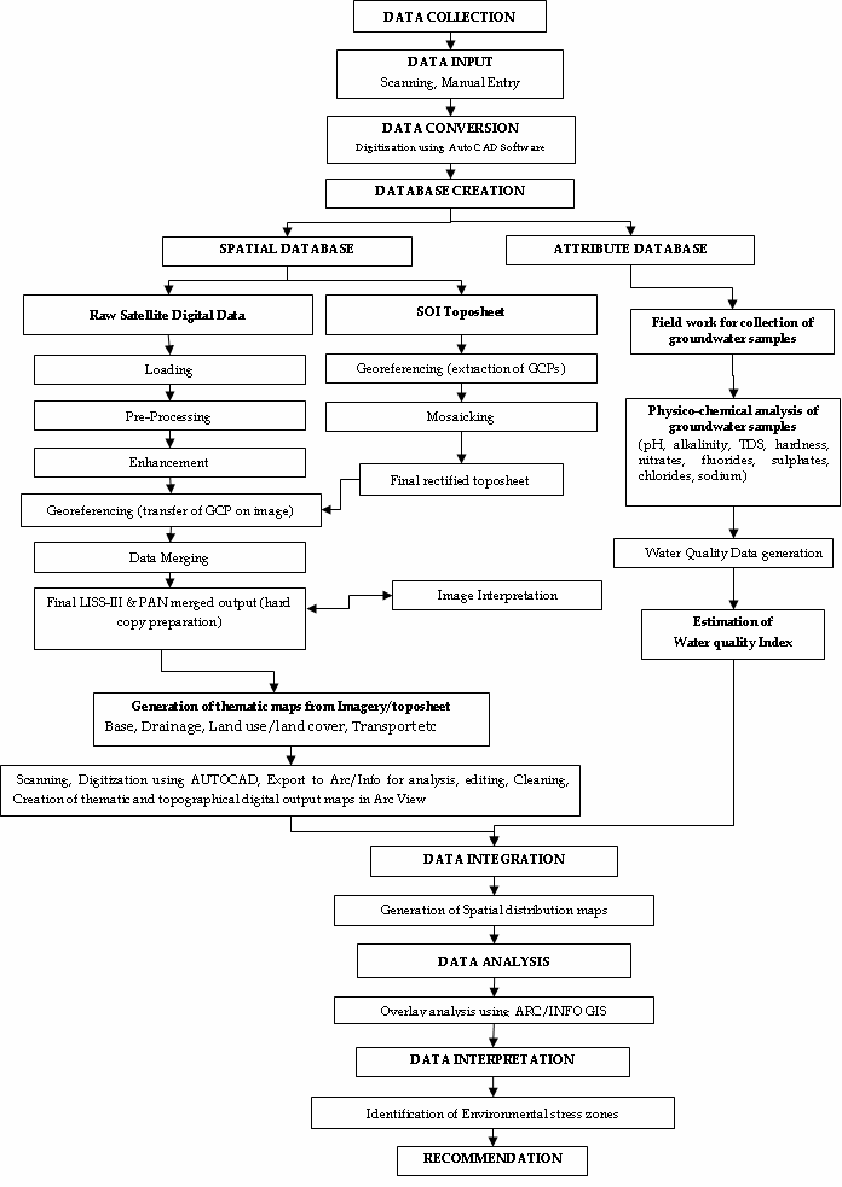
Spatial database
Thematic maps like base map and drainage network maps are prepared from the SOI toposheets on 1:50,000 scale using AutoCAD and Arc/Info GIS software to obtain a baseline data. All the maps are scanned and digitized to generate a digital output. Land use/Land cover map of the study area was prepared using visual interpretation technique from the fused satellite imagery (IRS-ID PAN + LISS-III) and SOI toposheets along with ground truth analysis.
Attribute database
Fieldwork was conducted and groundwater samples were collected from predetermined locations based on the land use change and drainage network maps of the study area. Map showing sampling points overlaid on satellite imagery as shown in Fig 2. The water samples were then analyzed for various physico-chemical parameters adopting standard protocols.
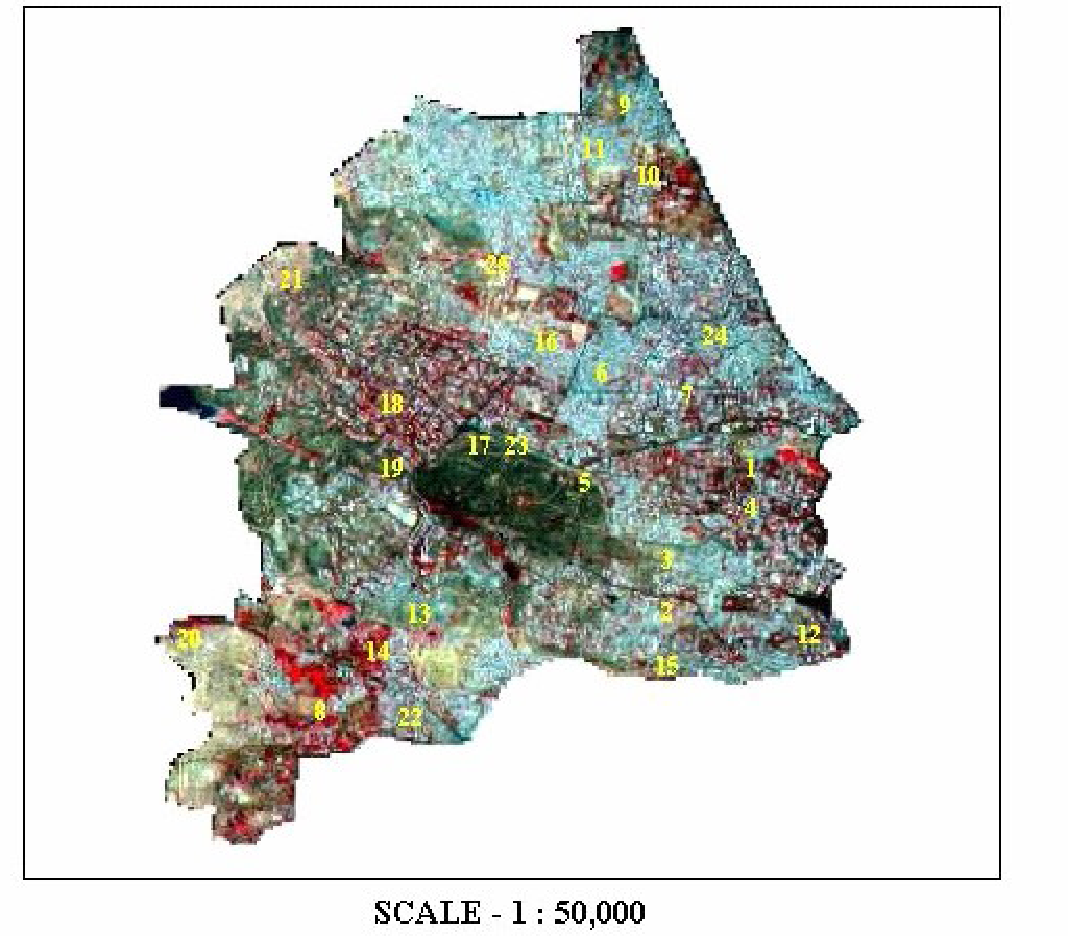
Integration of Spatial and Attribute Database
The spatial and the attribute database generated are integrated for the generation of spatial distribution maps of selected water quality parameters like pH, alkalinity, chlorides, sulphates, nitrates, TDS, total hardness, fluorides and Water Quality Index (WQI) and overlaid on satellite imagery. The water quality data (attribute) is linked to the sampling location (spatial) in ARC/INFO and maps showing spatial distribution are prepared to easily identify the variation in concentrations of the above parameters in the ground water at various locations of the study area using curve fitting technique of ARC/VIEW GIS software. Spatial Modelling and Surface Interpolation through IDW
GIS can be a powerful tool for developing solutions for water resources problems for assessing water quality, determining water availability, preventing flooding, understanding the natural environment, and managing water resources on a local or regional scale. Though there are a number of spatial modelling techniques available with respect to application in GIS, spatial interpolation technique through Inverse Distance Weighted (IDW) approach has been used in the present study to delineate the locational distribution of water pollutants or constituents. This method uses a defined or selected set of sample points for estimating the output grid cell value. It determines the cell values using a linearly weighted combination of a set of sample points and controls the significance of known points upon the interpolated values based upon their distance from the output point thereby generating a surface grid as well as thematic isolines.
Results and Discussion
The GIS-Based model in the present study uses logical conditioning and follows that of other models used in previous and similar studies (Chi & Lee, Krishnamurthy et al. 1996, Saraf et al., 1999 and Murthy, 2000). Before the thematic layers could be integrated with one another, each theme had to be weighted and scored. This relative ranking was based on pros and cons of the particular themes characteristic in terms of groundwater occurrence. The scores and weights ranged from
- 50 – Very good,
- 40 – Good,
- 30 – Moderate,
- 20 – Poor and
- 10 – Very poor.
For instance, an area that varies in landforms can be assigned various scores, such as for a level surface with unconsolidated material will be given a higher importance than an area with a steep impermeable slope. Similarly, areas that are characterised by more weathered /fractured geology is scored higher than that with compact and less weathered/fractured rock. After the categorisation, all the themes were converted to raster format using the ‘Spatial analyst’, tool in the ArcMap 9.2 software.
During the raster conversion, all the scores and weights assigned to the individual features were logged in the value field. In order to demarcate different groundwater potential zones, the six thematic layers were integrated according to their importance by a technique called ‘combinatorial method’. This is a type union function, whereby the function combines multiple rasters so that a unique output value is assigned to each unique combination of input values.
The names of the input rasters are assigned to the item names. Each of these items carries the unique input combination of values from the input rasters that produce the output value. These items retain the parentage that was used to produce the values for the output raster (ESRI, 2008). Finally by dividing theme weights by 100 based on importance normalized the individual themes, this was done instead of just dividing the maximum and minimum values into different categories. The outline for the combine sequence is shown in table 5.
In the first step, geology (L1) and the landforms (L2) layers were overlaid with one another to create the (R1) layer (Basically the resultant output of the two). The (R1) layer was then joined with the lineaments (L3) layers to produce (R2). The resultant layer of R2 was integrated with drainage density (L4) and so on until all six layers had been combined. The end product layer was that of (R5) which had a maximum score of 300 and a minimum of 60. Again the attribute values were checked and labelled in the final raster layer.
The final thematic map score values were then reclassified to show five classes of groundwater potential zones. These zones were very good, good, moderate, poor and very poor. Each of the five classes fell between an upper limit and a lower limits, this is shown in table 6.
The zones classed as ‘very good’ for groundwater potential fall between the upper limits of 300 and the lower limits of 240. Similarly the zones classed as ‘good’ were delineated between 240 and 180. This process was then repeated for the rest of the classes.
Table 5: Grouping of layers and total weights.
The final thematic map shows the study area with all the integrated layers. In the north, it indicates that the area in and around the structural hills, lineaments and mount complexes tends to have ‘very good to ‘good’ groundwater potential. Moving east along the north section of the map, it changes from ‘good’ to moderate where it reaches a Denudation hill in the northeast. Here the geology and slope alters from the Bokkeveld groups to TMS with a rise in elevation of around 500m to 1000m. There is also a decrease in the amount of lineaments in this section o the map. Focusing on the centre of the map and that mainly of the Rooiberg mountain range, groundwater potential starts to drop.
The predominant landforms here are Denudational structures with steep slopes and linear ridges. Here the geology is TMS with elongated, fractured and jointed features. These structures form much of the Rooiberg catchments area, valleys are present in-between them. Many of the valleys are situated on top of or around large substantial lineaments. The groundwater potential ranges from ‘poor’ to ‘very poor’ on the slopes of the Rooiberg and the high peaks of linear ridges.
However, the valleys offer ‘moderate’ to ‘good’ groundwater potential based on the drainage density and lineaments zone of influence. South of the Rooiberg the groundwater potential varies. Towards the southwest of the study area moving down from the Rooiberg into the lower formations of the Bokkeveld, the majority of the area is covered with residual hills with some structural hills and a residual mount complex farther south. Residual hills are far less spatially extensive than that of structural hills and the areas in-between these landforms are pediplains that are undulating surfaces consisting of broken up material of varying thickness.
These landscapes offer high permeability and porosity owing to the unconsolidated material. The groundwater potential here ranges from ‘moderate’ to ‘good’. In the southwest of the map, an alluvial plain that consist of Tertiary to Quaternary material (Le Roux, 1983) offers the potential to be ‘good to very good’ whereas the surrounding area is then a mixture of Wuppertal and Wagen Drift Bokkeveld formations dropping the potential from ‘poor to very poor’. Moving to the southeast section of the map, a mixture of potential ‘moderate to poor to very poor’ groundwater is present. Although there is a relatively high drainage percentage in this area, the geology varies from TMS to Bokkeveld mount complexes with the majority of these landforms having steep slopes.
The total area calculated for the groundwater potential zones were:
- Very Good – 124.4 km2,
- Good – 179.47 km2,
- Moderate – 114.77,
- Poor – 170.9 km2 and
- Very Poor – 92.9 km2.
Table 6: Upper and lower limits the final weights.
Module evaluation and field checks
Filed checks were carried out to ascertain the validity of the studies ‘groundwater model’. The model was checked against borehole and spring-seepage data (appendices), which reflects the status of the current groundwater potential. In total, seven borehole and two natural spring locations were recorded by acquiring their GPS coordinates, the local farmers gave the historic data for their own boreholes and springs. Other such data that was included in the observation was taken from groundwater abstraction test carried out on the Outspan farm in 2001 (21 15 17.77” E, 33 36 35.95”S) by the Ground Water Consulting Services. However, this data only reflects the groundwater potential to a certain extent in the study area and doses not represent all the groundwater potential ‘classes’.
The boreholes to the east and north of the Rooiberg are drilled to around 70m – 150m in the depth. Groundwater levels here are pumped at around 100m plus. The remaining four boreholes south of Rooiberg are drilled between 70m – 200m where the groundwater is pumped at depths ranging from 45m plus.
The amount of water that can be pumped is determined by the type of pumps been used. The majority of the pumps used in the region are solar pumps capable of pumping up to 14 000 Litres/24hr, depending on the depth. The pumps located to the north and east of the Rooiberg fall within the ‘moderate’ zone for potential groundwater and these are limited to pumping at 2 500 – 3 000 Litres/hr. The Outspan test (East Rooiberg) concluded that a maximum of 7 200 Litres/hr could be pumped within this zone (GCS, 2001). In comparison with the boreholes to the south of the mountain, the pumps are located within the ‘good’ zone on the map and the yield is around 1 400 – 5 000 Litres/hr.
The two springs are located south of the Rooiberg and are situated right against the mountain itself. The springs originate out from the TMS where there is a change in slope. Both are near large lineaments and valleys higher up in the mountain that suggest that any water there could contribute to the seepage. Both yielded in and around 500 Litres/hr, however this amount drops during dry periods.
Conclusion
Through this study, a methodological approach of using remote sensing and GIS techniques to demarcate groundwater potential zones was established. The preparation of different thematic maps that included geology, landforms, lineaments, drainage density, slope and elevation was derived from remote sensing data and by conventional methods (topographic maps and field checks). By integrating the thematic maps using logically conditions and GIS techniques of that used in previous studies, a final demarcation map of the east catchment section of the Rooiberg was produced. Field checks and yield data from borehole and springs were used to verify the validity of the model.
The study area showed that north of the catchment, prospect zones are demarcated as very good to good and moderate constituting about 40% of the mapped area. About 50% of the study area falls under the prospects zones of poor to very poor, apart from about 10% just south of the catchment area. Valleys fill, pediplains, the bases of structural and residual hills are found to be favourable geomorphic units for groundwater demarcation. Geomorphic units of complex mounts, residual mounts and linear ridges are found to be poor indicators for groundwater potential. However, slope, elevation, geology and lineaments play key roles in location of springs and groundwater. Thus the current study has shown, to an extent, the means of using remote sensing and GIS to demarcate groundwater potential in the semi-arid environment of the Rooiberg.
Further studies
Although the above study has demonstrated the capabilities of using remote sensing and GIS techniques as a platform in demarcating groundwater, the study can be refined and changed to include other geohydrological sources and techniques. Other sources of data such as maps of landuse/land cover, soils and weathered/fracture thickness zones can be incorporated into the GIS model and therefore improve the accuracy of determining the groundwater potential. Again, filed checks were only carried out in the ‘good to moderate’ zones of groundwater potential and so further investigation to the groundwater yield in the other classes should be carried out in validating the GIS model.
Other reliable tests that can be used include geophysical surveys and Vertical Electrical Sounding (VES) that produce geoelectrical profiles of the subsurface lithology and to an extent even water quality (Murthy, 1999). Other factors that could be included as further studies are the nature and extent of groundwater recharge. Although the current study revolved around delineating groundwater zones, further investigation should be carried out into understating the nature of aquifers and groundwater resources of the Rooiberg
References
Baron J, Seward P and Seymour A (1998). The groundwater harvest potential map of the Republic of South Africa. Technical report Gh3917 Dir. Geohdyrology, Dept of Water Affairs and Forestry, Pretoria.
Dept of Water Affairs (1986). Management of Water Resources of the Republic of South Africa.
Dept of Water Affairs & Forestry, 2002, A Groundwater Mapping Strategy for South Africa, Directorate Geohydrology publication, Pretoria, South Africa.
Dept of Water Affairs and Forestry (2004). Groundwater Use. Report 5E.
Dept of Water Affairs and Forestry (2005). Groundwater Quantification: Report 1D.
Dept of Water Affairs and Forestry (2005). Planning Potential Map: Report 2C.
Dept of Water Affairs and Forestry (2005). Recharge: Report 3aE.
Enslin, J.P., 1970. Die grondwaterpotensiaal van Suid-Afrika. Convention: Water for the future.
Girman J and Rosewarne P 2005. National Groundwater Resource Assessment Phase II. Biennial Groundwater Conference, Pretoria.
Haupt, C.J. 2001. Water Resources Situation Assessment. Directorate of Water Resource Planning. Dept of Water Affairs and Forestry. Groundwater Resources of South Africa. Compiled by WSM Engineers.
Rosewarne, P.N. 2005. Groundwater Use and Availability in South Africa. Biennial Groundwater Conference, Pretoria.
Vegter, J.R. (1995). An explanation of a set of national groundwater maps. Water Research Commission Report No TT 74/95, Pretoria, South Africa.
Vegter JR, Seymour AJ and Simonic M (1995). Groundwater resources of the Republic of South Africa. A set of 7 maps. Water Research Commission and Dept of Water Affairs and Forestry.
Vegter, J.R. 2001. Groundwater Development in South Africa and an Introduction to the Hydrogeology of Groundwater Regions. Water Research Commission. Report No TT134/00.